
- Chinese Optics Letters
- Vol. 20, Issue 1, 011701 (2022)
Abstract
1. Introduction
Abnormalities in eye perfusion have been linked to a number of sight-threatening diseases including glaucoma, retinal vein occlusions, diabetic retinopathy, age-related macular degeneration[
The disadvantage of conventional single-beam DOCT system is that flow velocity measurement depends on the Doppler angle between the incident beam and blood vessel orientation. Therefore, some researchers suggested using dual-beam detection systems to overcome this problem[
In this paper, we describe a dual-beam delay-encoded all fiber DOCT system (DDD-OCT) using only one spectrometer to measure the TRBF. Compared with Refs. [20,21], a beam displacer splits a beam of detection light into two probe beams in the sample arms that are delay-coded with different optical path lengths using a glass plate, which avoids the crosstalk between two channels. With this technique, only one spectrometer is required. A Dove prism[
Sign up for Chinese Optics Letters TOC. Get the latest issue of Chinese Optics Letters delivered right to you!Sign up now
2. Materials and Methods
Figure 1 shows the setup of the DDD-OCT. The light source is a low coherence superluminescent diode (SLD, Inphenix Inc.) with a central wavelength of 850 nm and an FWHM bandwidth of 50 nm. The lateral resolution is 22 µm, and the coherence length is 4.64 µm in tissue (
Figure 1.Schematic of the dual-beam delay-encoded fiber-based DOCT system. L1–L10, lens; L11, cylindrical lens; M1, mirror; M2, strip mirror; D1, D2, dichroic mirror; C1, C2, line scan camera; FC, fiber coupler; PC, polarization controller; F, fixation screen; GP, glass plate; BD, beam displacer; LD, 780 nm laser diode; LSLO, line scanning laser ophthalmoscopy system; SLD, superluminescent diode.
The LSLO system is used to provide real-time fundus preview imaging and guide the scanning position of OCT sampling beams, which is composed of a cylindrical lens (L11) that diverges the light beam from a 780 nm laser diode (LD) in a straight line, a strip gold mirror (M2) that isolates the entrance aperture from the exit aperture, and a line scan camera C2 (Atmel AVIIVA SM2 2014). The incident light power of the LSLO on the cornea is measured to be 1 mW, which is below the ANSI safety limit.
As shown in Fig. 2, the incident probe beams
Figure 2.Illustration of the incident probe beams and the blood flow velocity. V, direction of the blood flow velocity; P1 and P2, two probe beams; Δα, angle between the two probe beams; β, angle between V and the illumination plane (y–z plane, composed of P1 and P2).
Figure 3.Illustration of the illumination plane and scanning track. V, direction of the blood flow velocity; L, scanning track of OCT beams on the retina.
According to Eq. (1), the blood flow can be calculated as
3. Results
An in vitro experiment is carried out to verify our method. A capillary with an inner diameter of 135 µm, perfused with chicken blood at a constant flow velocity by a syringe pump, is used as the phantom sample. The capillary is immersed in diluted milk to suppress surface reflection. The calculation accuracy of the Doppler frequency shift depends on the sampling step between adjacent A-scans. In order to determine the optimized sampling step, the phantom flow at various sampling steps is measured to be compared with the set blood flow of 20 µL/min. As shown in Fig. 4(a), the accuracy of the measurement can be ensured with a sampling step less than 1.9 µm. In our experiment, the step spacing is chosen to be 1 µm. The measurement error of the system is within 10% [Fig. 4(b)], showing good accuracy and repeatability.
Figure 4.(a) Measured blood flow at the set blood flow of 20 µL/min as a function of the sampling step. (b) Measured blood flow versus the set blood flow.
To verify the system in vivo study, the blood flow in the retina of four healthy volunteers is imaged with the DDD-OCT system. This clinical study was approved by the Institutional Review Board of the Zhongshan Ophthalmic Center, Sun Yat-sen University, China and adhered to the tenets of the Declaration of Helsinki. The vessels with diameters above 60 µm are taken to calculate the TRBF. Informed consent is obtained from the subject before the experiment.
The probe beams are scanned around the ONH, as shown in Fig. 5(a), to determine the TRBF. The structural and DOCT images of the artery vessel
Figure 5.Retinal blood flow imaging with the DDD-OCT system. (a) LSLO fundus view. The black line marks the scanning track. V1, an artery vessel. (b) Structural and (c) Doppler OCT images of the vessel V1. (d) Absolute velocity of the blood flow in V1 as a function of time.
The determination of vessel diameter is critical for calculating the blood flow. To minimize the impact of the low phase difference at the border of the vessel, we select the OCT phase image near a systolic peak to extract the vessel diameter[
4. Discussion and Conclusions
In previously published reports by Riva et al.[
Our study has several limitations, including the angular separation between both beams, which cannot be adjusted at will. Secondly, for the poor fixation subjects, our system could not measure their blood flow. To overcome this difficulty, eye-tracking technology is needed. Finally, the vascular outlines are delineated by a human expert, which has certain subjectivity. A more objective boundary delineating software is needed.
In conclusion, we described a beam-displacer-based DDD-OCT system to accurately calculate the blood flow over the cardiac cycle. With two probe beams delay-coded with different optical path lengths, the DDD-OCT system is capable of blood flow imaging in both directions at the same time without crosstalk between two channels, as compared with Refs. [20,21]. The integrated unit of LSLO makes the acquisition process more intuitive and convenient. The in vivo study demonstrates the ability of the DDD-OCT to measure the retinal blood flow, which offers promising potential for monitoring retinal blood flow abnormalities in patients.
References
[1] T. A. Ciulla, A. Harris, P. Latkany, H. C. Piper, O. Arend, H. Garzozi, B. Martin. Ocular perfusion abnormalities in diabetes. Acta. Ophthalmol., 80, 468(2002).
[2] A. Harris, H. S. Chung, T. A. Ciulla, L. Kagemann. Progress in measurement of ocular blood flow and relevance to our understanding of glaucoma and age-related macular degeneration. Prog. Retin. Eye Res., 18, 669(1999).
[3] J. C. Hwang, R. Konduru, X. Zhang, O. Tan, B. A. Francis, R. Varma, M. Sehi, D. S. Greenfield, S. R. Sadda, D. Huang. Relationship among visual field, blood flow, and neural structure measurements in glaucoma. Invest. Ophthalmol. Vis. Sci., 53, 3020(2012).
[4] D. Huang, E. A. Swanson, C. P. Lin, J. S. Schuman, W. G. Stinson, W. Chang, M. R. Hee, T. Flotte, K. Gregory, C. A. Puliafito, J. G. Fujimoto. Optical coherence tomography. Science, 254, 1178(1991).
[5] X. Chen, Y. Lei, Y. Wang, D. Yu. Resolution enhancement with improved range Doppler algorithm in high numerical aperture OCT. Chin. Opt. Lett., 9, 121001(2011).
[6] D. C. Adler, T. H. Ko, J. G. Fujimoto. Speckle reduction in optical coherence tomography images by use of a spatially adaptive wavelet filter. Opt. Lett., 29, 2878(2004).
[7] P. M. Andrews, H. W. Wang, J. Wierwille, W. Gong, J. Verbesey, M. Cooper, Y. Chen. Optical coherence tomography of the living human kidney. J. Innov. Opt. Heal. Sci., 07, 1350064(2014).
[8] J. Q. Dong, Q. H. Li, Y. Q. Hu. Multi-technique analysis of an ancient stratified glass eye bead by OCT, µ-XRF, and µ-Raman spectroscopy. Chin. Opt. Lett., 18, 090001(2020).
[9] S. Wang, J. Zhou, J. F. Jiang, K. Liu, Q. Han, Y. N. Duan, R. D. Wang, T. G. Liu. Multi-channel polarized low-coherence interference synchronous demodulation system based on a matrix charge-coupled device. Chin. Opt. Lett., 18, 071202(2020).
[10] L. Huang, Y. M. Fu, R. X. Chen, S. S. Yang, H. X. Qiu, X. Wu, S. Y. Zhao, Y. Gu, P. Li. SNR-adaptive OCT angiography enabled by statistical characterization of intensity and decorrelation with multi-variate time series model. IEEE Trans. Med. Imaging, 38, 2695(2019).
[11] H. K. Li, K. Y. Liu, T. T. Cao, L. Yao, Z. Y. Zhang, X. F. Deng, C. X. Du, P. Li. High performance OCTA enabled by combining features of shape, intensity, and complex decorrelation. Opt. Lett., 46, 368(2021).
[12] M. Bonesi, A. J. Kennerley, I. Meglinski, S. Matcher. Application of Doppler optical coherence tomography in rheological studies: blood flow and vessels mechanical properties evaluation. J. Innov. Opt. Heal. Sci., 2, 431(2009).
[13] O. V. Semyachkina-Glushkovskaya, V. V. Lychagov, O. A. Bibikova, I. A. Semyachkin-Glushkovskiy, S. S. Sindeev, E. M. Zinchenko, M. M. Kassim, A.-F. fatema Ali, A. H. Leith, M. V. Ulanova, V. V. Tuchin. The experimental study of stress-related pathological changes in cerebral venous blood flow in newborn rats assessed by doct. J. Innov. Opt. Heal. Sci., 6, 1350023(2013).
[14] G. Liu, Z. Chen. Advances in Doppler OCT. Chin. Opt. Lett., 11, 011702(2013).
[15] R. Dsouza, H. Subhash, K. Neuhaus, P. M. McNamara, J. Hogan, C. Wilson, M. J. Leahy. Feasibility study of phase-sensitive imaging based on multiple reference optical coherence tomography. Chin. Opt. Lett., 15, 090007(2017).
[16] R. A. Leitgeb, R. M. Werkmeister, C. Blatter, L. Schmetterer. Doppler optical coherence tomography. Prog. Retin. Eye. Res., 41, 26(2014).
[17] D. P. Dave, T. E. Milner. Doppler-angle measurement in highly scattering media. Opt. Lett., 25, 1523(2000).
[18] G. C. Aschinger, L. Schmetterer, V. Doblhoff-Dier, R. A. Leitgeb, G. Garhöfer, M. Gröschl, R. M. Werkmeister. Blood flow velocity vector field reconstruction from dual-beam bidirectional Doppler OCT measurements in retinal veins. Biomed. Opt. Express, 6, 1599(2015).
[19] V. Doblhoff-Dier, L. Schmetterer, W. Vilser, G. Garhöfer, M. Gröschl, R. A. Leitgeb, R. M. Werkmeister. Measurement of the total retinal blood flow using dual beam Fourier-domain Doppler optical coherence tomography with orthogonal detection planes. Biomed. Opt. Express, 5, 630(2014).
[20] C. J. Pedersen, D. Huang, M. A. Shure, A. M. Rollins. Measurement of absolute flow velocity vector using dual-angle, delay-encoded Doppler optical coherence tomography. Opt. Lett., 32, 506(2007).
[21] L. M. Peterson, S. Gu, M. W. Jenkins, A. M. Rollins. Orientation-independent rapid pulsatile flow measurement using dual-angle Doppler OCT. Biomed. Opt. Express, 5, 499(2014).
[22] R. Michaely, A. H. Bachmann, M. L. Villiger, C. Blatter, T. Lasser, R. A. Leitgeb. Vectorial reconstruction of retinal blood flow in three dimensions measured with high resolution resonant Doppler Fourier domain optical coherence tomography. J. Biomed. Opt., 12, 041213(2007).
[23] S. Makita, T. Fabritius, Y. Yasuno. Quantitative retinal-blood flow measurement with three-dimensional vessel geometry determination using ultrahigh-resolution Doppler optical coherence angiography. Opt. Lett., 33, 836(2008).
[24] B. Baumann, B. Potsaid, M. F. Kraus, J. J. Liu, D. Huang, J. Hornegger, A. E. Cable, J. S. Duker, J. G. Fujimoto. Total retinal blood flow measurement with ultrahigh speed swept source/Fourier domain OCT. Biomed. Opt. Express, 2, 1539(2011).
[25] W. Choi, B. Baumann, J. J. Liu, A. C. Clermont, E. P. Feener, J. S. Duker, J. G. Fujimoto. Measurement of pulsatile total blood flow in the human and rat retina with ultrahigh speed spectral/Fourier domain OCT. Biomed. Opt. Express, 3, 1047(2012).
[26] Y. Wang, B. A. Bower, J. A. Izatt, O. Tan, D. Huang. In vivo retinal blood flow measurement by Fourier domain Doppler optical coherence tomography. J. Biomed. Opt., 12, 041215(2007).
[27] Y. Wang, B. A. Bower, J. A. Izatt, O. Tan, D. Huang. Retinal blood flow measurement by circumpapillary Fourier domain Doppler optical coherence tomography. J. Biomed. Opt., 13, 064003(2008).
[28] W. Trasischker, R. M. Werkmeister, S. Zotter, B. Baumann, T. Torzicky, M. Pircher, C. K. Hitzenberger. In vitro and in vivo three-dimensional velocity vector measurement by three-beam spectral-domain Doppler optical coherence tomography. J. Biomed. Opt., 18, 116010(2013).
[29] R. Haindl, W. Trasischker, A. Wartak, B. Baumann, M. Pircher, C. K. Hitzenberger. Total retinal blood flow measurement by three beam Doppler optical coherence tomography. Biomed. Opt. Express, 7, 287(2016).
[30] A. Wartak, R. Haindl, W. Trasischker, B. Baumann, M. Pircher, C. K. Hitzenberger. Active-passive path-length encoded (APPLE) Doppler OCT. Biomed. Opt. Express, 7, 5233(2016).
[31] A. Wartak, F. Beer, B. Baumann, M. Pircher, C. K. Hitzenberger. Adaptable switching schemes for time-encoded multichannel optical coherence tomography. J. Biomed. Opt., 23, 056010(2018).
[32] C. Blatter, S. Coquoz, B. Grajciar, A. S. G. Singh, M. Bonesi, R. M. Werkmeister, L. Schmetterer, R. A. Leitgeb. Dove prism based rotating dual beam bidirectional Doppler OCT. Biomed. Opt. Express, 4, 1188(2013).
[33] R. M. Werkmeister, N. Dragostinoff, M. Pircher, E. Götzinger, C. K. Hitzenberger, R. A. Leitgeb, L. Schmetterer. Bidirectional Doppler Fourier-domain optical coherence tomography for measurement of absolute flow velocities in human retinal vessels. Opt. Lett., 33, 2967(2008).
[34] S. Norrby, P. Piers, C. Campbell, M. V. D. Mooren. Model eyes for evaluation of intraocular lenses. Appl. Opt., 46, 6595(2007).
[35] C. Blatter, B. Grajciar, L. Schmetterer, R. A. Leitgeb. Angle independent flow assessment with bidirectional Doppler optical coherence tomography. Opt. Lett., 38, 4433(2013).
[36] C. Kolbitsch, T. Schmoll, R. A. Leitgeb. Histogram-based filtering for quantitative 3D retinal angiography. J. Biophotonics, 2, 416(2009).
[37] C. E. Riva, J. E. Grunwald, S. H. Sinclair, B. L. Petrig. Blood velocity and volumetric flow rate in human retinal vessels. Invest. Ophthalmol. Vis. Sci., 26, 1124(1985).
[38] Y. Wang, A. Lu, J. Gil-Flamer, O. Tan, J. A. Izatt, D. Huang. Measurement of total blood flow in the normal human retina using Doppler Fourier-domain optical coherence tomography. Br. J. Ophthalmol., 93, 634(2009).
[39] Y. Wang, A. A. Fawzi, R. Varma, A. A. Sadun, X. Zhang, O. Tan, J. A. Izatt, D. Huang. Pilot study of optical coherence tomography measurement of retinal blood flow in retinal and optic nerve diseases. Invest. Ophthalmol. Vis. Sci., 52, 840(2011).
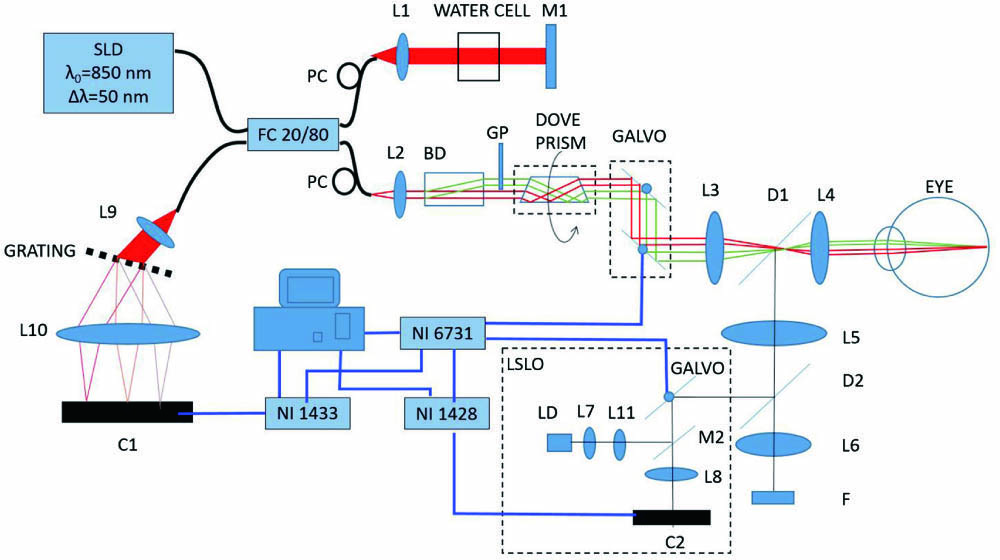
Set citation alerts for the article
Please enter your email address