
- Chinese Optics Letters
- Vol. 20, Issue 3, 031302 (2022)
Abstract
1. Introduction
Silicon carbide (SiC), especially the 4H polytype, is a promising platform for realizing large-scale integrated photonics owing to its prominent material properties, such as high refractive index (2.6 at the C-band), large transparent window, high second- and third-order optical nonlinearities[
A central challenge of 4H-SiC for spin-based quantum information applications lies in the ability to efficiently extract photoluminescence (PL) emitted by the optically addressable spin defects and to simultaneously engineer the two-level solid-state quantum emitters with a high fidelity[
In this work, we design and fabricate 1D-PhC cavities on a 4H-SiCOI platform prepared by the ion-cutting method. We characterize the fabricated PhC cavities using a room temperature PL experiment. The measured PL spectra unveil that the nanophotonic cavities supported transverse-electric (TE)-like modes. The fundamental TE (
Sign up for Chinese Optics Letters TOC. Get the latest issue of Chinese Optics Letters delivered right to you!Sign up now
2. Device Design and Fabrication
The 4H-SiCOI used in this work was prepared by the ion-cutting technique, together with a direct wafer bonding technique. The detailed fabrication process is depicted elsewhere[
As for the device processing, an 800 nm thick H silsesquioxane (HSQ) resist was spin-coated on top of the 4H-SiCOI substrate, and the PhC nanobeam patterns were defined via an electron-beam lithography (EBL) process. The patterns were then transferred to the 4H-SiC film via ICP etching with
Figure 1.(a) Scanning electron microscope image of a suspended 1D nanobeam PhC cavity. (b) Zoom-in side view image of the PhC cavity, where th is the slab thickness. (c) Zoom-in top view image of the 1D-PhC cavity. The nominal unit cell is parameterized by a (lattice constant, hole to hole distance), w (beam width), hx (air hole length), and hy(air hole width).
3. Result and Simulation
To characterize the resonance properties of the fabricated 1D-PhC cavities, we measured the PL spectra of the devices at room temperature using a home-built µ-PL setup, as depicted in the previous work[
An experimental PL spectrum of the PhC nanobeam cavity is shown (blue line) in Fig. 2(a). The broad emission background is likely attributed to lattice imperfections and oxygen-related surface defects introduced by the proton implantation and device fabrication process, which was extensively observed in SiC PL measurement[
Figure 2.(a) Experimentally measured PL spectrum (blue line) and 3D-FDTD simulation spectrum (purple line) from a PhC with a lattice constant of 290 nm. Resonance peaks in the simulated spectrum show a good consistence with the experimental results at room temperature, especially the TE-like polarized modes. (b)–(d) 3D finite-element-method (FEM) simulated optical profiles (Ey component) of the (b) TE0, (c) TE1, and (d) TE2 modes.
Next, we characterize the geometry-dependent cavity properties. Figure 3(a) shows the normalized PL spectra of the cavities with different lattice constants. The fundamental
Figure 3.(a) PL spectra of 1D-PhC cavities with lattice constants of 270 nm and 290 nm. The fundamental TE-like modes are located at 753.8 nm and 825.4 nm, respectively. (b), (c) Normalized PL spectra and theoretical fitting (red lines) of the TE0 modes for cavities with lattice constants of (b) 270 nm and (c) 290 nm.
In addition, we evaluate another critical parameter of the PhC cavities, i.e., the mode volume (
Given the calculated mode volume
In order to overcome inherent variations of the resonant wavelengths and the inhomogeneous broadening of the spin defects in 4H-SiC, a critical step for realizing an efficient cavity-defect system will be the demonstration of spectral tuning of cavity modes. So far, various techniques such as cryogenic gas condensation[
Figure 4.(a) Measured PL spectra as a function of the excitation power. (b) Resonance shift of the TE-like modes (rectangle for TE0, circle for TE2, triangle for TE3) as a function of excitation power.
4. Conclusion
In conclusion, we have demonstrated 1D-PhC nanobeam cavities on an ion-cutting 4H-SiCOI platform. The cavity modes are located in the visible and near-infrared wavelength range. The cavity shows experimental
References
[1] B.-S. Song, T. Asano, S. Jeon, H. Kim, C. Chen, D. D. Kang, S. Noda. Ultrahigh-Q photonic crystal nanocavities based on 4H silicon carbide. Optica, 6, 991(2019).
[2] C. Wang, Z. Fang, A. Yi, B. Yang, Z. Wang, L. Zhou, C. Shen, Y. Zhu, Y. Zhou, R. Bao, Z. Li, Y. Chen, K. Huang, J. Zhang, Y. Cheng, X. Ou. High-Q microresonators on 4H-silicon-carbide-on-insulator platform for nonlinear photonics. Light Sci. Appl., 10, 139(2021).
[3] H. Sato, M. Abe, I. Shoji, J. Suda, T. Kondo. Accurate measurements of second-order nonlinear optical coefficients of 6H and 4H silicon carbide. J. Opt. Soc. Am. B, 26, 1892(2009).
[4] Y. Zheng, M. Pu, A. Yi, X. Ou, H. Ou. 4H-SiC microring resonators for nonlinear integrated photonics. Opt. Lett., 44, 5784(2019).
[5] M. A. Guidry, K. Y. Yang, D. M. Lukin, A. Markosyan, J. Yang, M. M. Fejer, J. Vučković. Optical parametric oscillation in silicon carbide nanophotonics. Optica, 7, 1139(2020).
[6] S. Castelletto, A. Boretti. Silicon carbide color centers for quantum applications. J. Phys., 2, 022001(2020).
[7] M. Atatüre, D. Englund, N. Vamivakas, S.-Y. Lee, J. Wrachtrup. Material platforms for spin-based photonic quantum technologies. Nat. Rev. Mater., 3, 38(2018).
[8] S. Castelletto, B. C. Johnson, V. Ivady, N. Stavrias, T. Umeda, A. Gali, T. Ohshima. A silicon carbide room-temperature single-photon source. Nat. Mater., 13, 151(2014).
[9] D. J. Christle, A. L. Falk, P. Andrich, P. V. Klimov, J. U. Hassan, N. T. Son, E. Janzén, T. Ohshima, D. D. Awschalom. Isolated electron spins in silicon carbide with millisecond coherence times. Nat. Mater., 14, 160(2015).
[10] D. M. Lukin, C. Dory, M. A. Guidry, K. Y. Yang, S. D. Mishra, R. Trivedi, M. Radulaski, S. Sun, D. Vercruysse, G. H. Ahn, J. Vučković. 4H-silicon-carbide-on-insulator for integrated quantum and nonlinear photonics. Nat. Photonics, 14, 330(2019).
[11] D. D. Awschalom, R. Hanson, J. Wrachtrup, B. B. Zhou. Quantum technologies with optically interfaced solid-state spins. Nat. Photonics, 12, 516(2018).
[12] B. Lienhard, T. Schröder, S. Mouradian, F. Dolde, T. T. Tran, I. Aharonovich, D. Englund. Bright and photostable single-photon emitter in silicon carbide. Optica, 3, 768(2016).
[13] N. Morioka, C. Babin, R. Nagy, I. Gediz, E. Hesselmeier, D. Liu, M. Joliffe, M. Niethammer, D. Dasari, V. Vorobyov, R. Kolesov, R. Stohr, J. Ul-Hassan, N. T. Son, T. Ohshima, P. Udvarhelyi, G. Thiering, A. Gali, J. Wrachtrup, F. Kaiser. Spin-controlled generation of indistinguishable and distinguishable photons from silicon vacancy centres in silicon carbide. Nat. Commun., 11, 2516(2020).
[14] M. Widmann, S.-Y. Lee, T. Rendler, N. T. Son, H. Fedder, S. Paik, L.-P. Yang, N. Zhao, S. Yang, I. Booker, A. Denisenko, M. Jamali, S. A. Momenzadeh, I. Gerhardt, T. Ohshima, A. Gali, E. Janzén, J. Wrachtrup. Coherent control of single spins in silicon carbide at room temperature. Nat. Mater., 14, 164(2014).
[15] R. Nagy, M. Niethammer, M. Widmann, Y. C. Chen, P. Udvarhelyi, C. Bonato, J. U. Hassan, R. Karhu, I. G. Ivanov, N. T. Son, J. R. Maze, T. Ohshima, O. O. Soykal, A. Gali, S. Y. Lee, F. Kaiser, J. Wrachtrup. High-fidelity spin and optical control of single silicon-vacancy centres in silicon carbide. Nat. Commun., 10, 1954(2019).
[16] K. Sakoda, T. Kuroda, N. Ikeda, T. Mano, Y. Sugimoto, T. Ochiai, K. Kuroda, S. Ohkouchi, N. Koguchi, K. Asakawa. Purcell effect of GaAs quantum dots by photonic crystal microcavities. Chin. Opt. Lett., 7, 879(2009).
[17] L. Fang, X. Gan, J. Zhao. High-Q factor photonic crystal cavities with cut air holes [Invited]. Chin. Opt. Lett., 18, 111402(2020).
[18] A. Sipahigil, R. E. Evans, D. D. Sukachev, M. J. Burek, J. Borregaard, M. K. Bhaskar, C. T. Nguyen, J. L. Pacheco, H. A. Atikian, C. Meuwly, R. M. Camacho, F. Jelezko, E. Bielejec, H. Park, M. Loncar, M. D. Lukin. An integrated diamond nanophotonics platform for quantum-optical networks. Science, 354, 847(2016).
[19] A. L. Crook, C. P. Anderson, K. C. Miao, A. Bourassa, H. Lee, S. L. Bayliss, D. O. Bracher, X. Zhang, H. Abe, T. Ohshima, E. L. Hu, D. D. Awschalom. Purcell enhancement of a single silicon carbide color center with coherent spin control. Nano Lett., 20, 3427(2020).
[20] J. L. Zhang, S. Sun, M. J. Burek, C. Dory, Y. K. Tzeng, K. A. Fischer, Y. Kelaita, K. G. Lagoudakis, M. Radulaski, Z. X. Shen, N. A. Melosh, S. Chu, M. Loncar, J. Vuckovic. Strongly cavity-enhanced spontaneous emission from silicon-vacancy centers in diamond. Nano Lett., 18, 1360(2018).
[21] R. Ge, X. Yan, Y. Chen, X. Chen. Broadband and lossless lithium niobate valley photonic crystal waveguide [Invited]. Chin. Opt. Lett., 19, 060014(2021).
[22] A. Yi, Y. Zheng, H. Huang, J. Lin, Y. Yan, T. You, K. Huang, S. Zhang, C. Shen, M. Zhou, W. Huang, J. Zhang, S. Zhou, H. Ou, X. Ou. Wafer-scale 4H-silicon carbide-on-insulator (4H–SiCOI) platform for nonlinear integrated optical devices. Opt. Mater., 107, 109990(2020).
[23] C. Wang, C. Shen, A. Yi, S. Yang, L. Zhou, Y. Zhu, K. Huang, S. Song, M. Zhou, J. Zhang, X. Ou. Visible and near-infrared microdisk resonators on a 4H-silicon-carbide-on-insulator platform. Opt. Lett., 46, 2952(2021).
[24] Y. Zheng, M. Pu, A. Yi, B. Chang, T. You, K. Huang, A. N. Kamel, M. R. Henriksen, A. A. Jørgensen, X. Ou, H. Ou. High-quality factor, high-confinement microring resonators in 4H-silicon carbide-on-insulator. Opt. Express, 27, 13053(2019).
[25] J. Chan, M. Eichenfield, R. Camacho, O. Painter. Optical and mechanical design of a “zipper” photonic crystal optomechanical cavity. Opt. Express, 17, 3802(2009).
[26] M. Eichenfield, J. Chan, R. M. Camacho, K. J. Vahala, O. Painter. Optomechanical crystals. Nature, 462, 78(2009).
[27] M. Radulaski, T. M. Babinec, K. Müller, K. G. Lagoudakis, J. L. Zhang, S. Buckley, Y. A. Kelaita, K. Alassaad, G. Ferro, J. Vučković. Visible photoluminescence from cubic (3C) silicon carbide microdisks coupled to high quality whispering gallery modes. ACS Photonics, 2, 14(2014).
[28] S. Castelletto, A. F. M. Almutairi, K. Kumagai, T. Katkus, Y. Hayasaki, B. C. Johnson, S. Juodkazis. Photoluminescence in hexagonal silicon carbide by direct femtosecond laser writing. Opt. Lett., 43, 6077(2018).
[29] M. Rühl, C. Ott, S. Götzinger, M. Krieger, H. B. Weber. Controlled generation of intrinsic near-infrared color centers in 4H-SiC via proton irradiation and annealing. Appl. Phys. Lett., 113, 122102(2018).
[30] S. Wang, M. Zhan, G. Wang, H. Xuan, W. Zhang, C. Liu, C. Xu, Y. Liu, Z. Wei, X. Chen. 4H-SiC: a new nonlinear material for midinfrared lasers. Laser Photonics Rev., 7, 831(2013).
[31] M. N. Gadalla, A. S. Greenspon, R. K. Defo, X. Zhang, E. L. Hu. Enhanced cavity coupling to silicon vacancies in 4H silicon carbide using laser irradiation and thermal annealing. Proc. Natl. Acad. Sci. U. S. A., 118, e2021768118(2021).
[32] A. Faraon, P. E. Barclay, C. Santori, K.-M. C. Fu, R. G. Beausoleil. Resonant enhancement of the zero-phonon emission from a colour centre in a diamond cavity. Nat. Photonics, 5, 301(2011).
[33] E. Burstein, E. M. Purcell, C. Weisbuch. Spontaneous emission probabilities at radio frequencies. Confined Electrons and Photons: New Physics and Applications, 839(1995).
[34] A. Lohrmann, T. J. Karle, V. K. Sewani, A. Laucht, M. Bosi, M. Negri, S. Castelletto, S. Prawer, J. C. McCallum, B. C. Johnson. Integration of single-photon emitters into 3C-SiC microdisk resonators. ACS Photonics, 4, 462(2017).
[35] S. Kiravittaya, H. S. Lee, L. Balet, L. H. Li, M. Francardi, A. Gerardino, A. Fiore, A. Rastelli, O. G. Schmidt. Tuning optical modes in slab photonic crystal by atomic layer deposition and laser-assisted oxidation. J. Appl. Phys., 109, 053115(2011).
[36] X. Wu, T. Fan, A. A. Eftekhar, A. Adibi. High-Q microresonators integrated with microheaters on a 3C-SiC-on-insulator platform. Opt. Lett., 44, 4941(2019).
[37] X. Y. Lu, J. Y. Lee, P. X. L. Feng, Q. Lin. Silicon carbide microdisk resonator. Opt. Lett., 38, 1304(2013).
[38] J. C. Lee, D. O. Bracher, S. Cui, K. Ohno, C. A. McLellan, X. Zhang, P. Andrich, B. Alemán, K. J. Russell, A. P. Magyar, I. Aharonovich, A. Bleszynski Jayich, D. Awschalom, E. L. Hu. Deterministic coupling of delta-doped nitrogen vacancy centers to a nanobeam photonic crystal cavity. Appl. Phys. Lett., 105, 261101(2014).
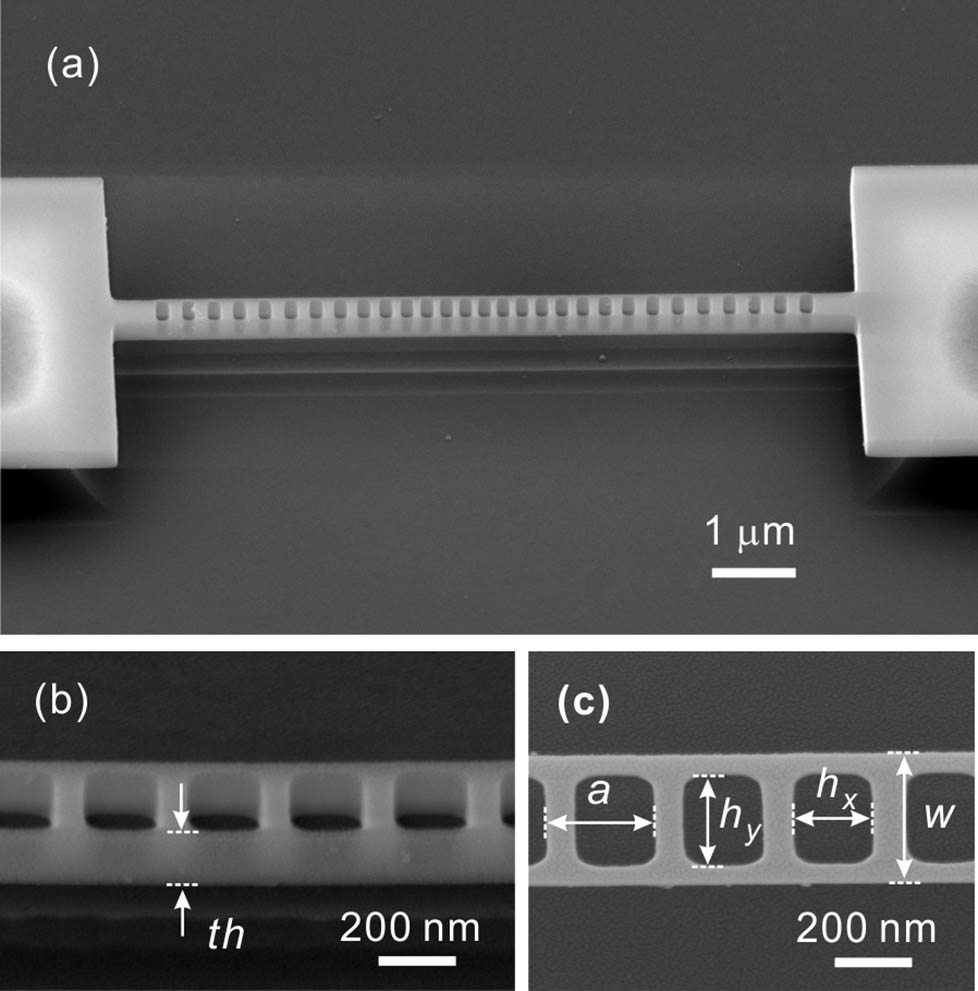
Set citation alerts for the article
Please enter your email address