
- Photonics Research
- Vol. 10, Issue 6, 1401 (2022)
Abstract
1. INTRODUCTION
Optical phase control is a key functionality to modulate optical signals in photonic integrated circuits (PICs) for various applications. In particular, integrated phase modulator-based micro-ring resonators (MRRs) are becoming increasingly important due to their compact footprint, high Q-factor, and low cost [1–4]. In contrast to the traditional silicon on insulator (SOI), the silicon nitride (SiN) platform has stimulated great interest because of its ultralow propagation loss and broadband transparency window. Moreover, a thick SiN platform is expected for high optical confinement and dispersion engineering to enhance nonlinear optical properties [5,6]. The MRR-based on-chip modulator as a most important device for the optical link shows great potential in the field of high-volume optical signal processing [7–11]. The optical signal can be effectively controlled through engineering the effective index of the optical waveguide. State-of-the-art silicon modulators rely on the phase modulation through the plasma dispersion effect, either by injection, accumulation, or depletion of free carrier to control the effective index of the waveguide. Despite being relatively fast and efficient, these devices suffer from a complex fabrication process and high insertion loss [12]. Alternative approaches are based on heterogeneous integration with materials such as III-V semiconductors, electro-optic organic layers, or epitaxial barium titanate (BTO) [13–17]. However, most of the above-mentioned solutions are not feasible using the SiN platform. Specifically, thanks to its insulating nature (
In this work, we fabricate a composite
2. RESULTS AND DISCUSSION
We deposited 3 μm thermally oxidized
Sign up for Photonics Research TOC. Get the latest issue of Photonics Research delivered right to you!Sign up now
Figure 1.(a) Schematic of a few-layer
As shown in Fig. 2(a), the broadband linear absorption spectrum of few-layer
Figure 2.(a) Broadband linear absorption spectrum of few-layer
The bias voltage is applied across the two electrodes to achieve electrostatic doping of the
Figure 3.(a) Normalized transmission response of the phase shifter as a function of applied bias voltage (cations doping on the interface of
Figure 4.(a) Normalized transmission response of a phase shifter as a function of applied bias voltage (anion doping on the surface of
Figure 5.(a) Normalized transmission response of a phase shifter at high bias voltages. (b) The offset of resonance wavelength loses its linearity at
Interestingly, besides the phase modulation, the output spectra under different types of charge doping also display regular intensity modulation, which is desired for enormous applications such as optical frequency combs and microwave photonics. Unlike the monolayer
Figure 6.The effective index of the composite
In Table 1, we compare the performance of the present hybrid integrated device with the state-of-the-art, including silicon–graphene-hybrid MZMs, silicon–
Comparison of Phase Tuning Efficiency for the Hybrid Integrated Phase Modulator
Platform | Structure | Method | Cross Section of Waveguide | Insertion Loss (dB) | Refs. | |
---|---|---|---|---|---|---|
SOI | MZM | Graphene–silicon | / | 0.28 | [ | |
SOI | MZM | 2.5 | 2.2 | [ | ||
SOI | MZM | Organic–silicon | 5.4 | 1.4 | [ | |
SOI | MZM | ITO–silicon | 6.7 | 0.0095 | [ | |
SiN | MRR | PZT–SiN | / | 3.3 | [ | |
SiN | Racetrack | 13 | 5.1 | [ | ||
SiN | MZM | 1.7 | [ | |||
MRR | / | 0.8 | ||||
SiN | MRR | 3.2 | 0.69 | This work |
MZM, Mach–Zehnder modulator; PZT, piezoelectric lead zirconate titanate; Racetrack, racetrack resonator.
3. CONCLUSION
We have experimentally demonstrated a
References
[1] S. Brian, X. C. Ji, Y. Okawachi, A. L. Gaeta, M. Lipson. Battery-operated integrated frequency comb generator. Nature, 562, 401-405(2018).
[2] M. La Notte, B. Troia, T. Muciaccia, C. E. Campanella, F. De Leonardis, V. M. N. Passaro. Recent advances in gas and chemical detection by Vernier effect-based photonic sensors. Sensors, 14, 4831-4855(2014).
[3] Y. Tao, H. Shu, X. Wang, M. Jin, Z. Tao, F. Yang, J. Shi, J. Qin. Hybrid-integrated high-performance microwave photonic filter with switchable response. Photon. Res., 9, 1569-1580(2021).
[4] X. Xue, X. Zheng, B. Zhou. Super-efficient temporal solitons in mutually coupled optical cavities. Nat. Photonics, 13, 616-622(2019).
[5] Y. Okawachi, M. Lamont, K. Luke, D. Carvalho, M. Yu, M. Lipson, A. L. Gaeta. Bandwidth shaping of microresonator-based frequency combs via dispersion engineering. Opt. Lett., 39, 3535-3538(2014).
[6] Y. Zhao, X. Ji, B. Y. Kim, P. S. Donvalkar, J. K. Jang, C. Joshi, M. Yu, C. Joshi, R. R. Domeneguetti, F. A. S. Barbosa, P. Nussenzveig, Y. Okawachi, M. Lipson, A. L. Gaeta. Visible nonlinear photonics via high-order-mode dispersion engineering. Optica, 7, 135-141(2020).
[7] S. Yang, D. C. Liu, Z. L. Tan, K. Liu, Z. H. Zhu, S. Q. Qin. CMOS-compatible WS2-based all-optical modulator. ACS Photon., 5, 342-346(2018).
[8] H. Lin, Z. Luo, T. Gu, L. C. Kimerling, K. Wada, A. Agarwal, J. Hu. Mid-infrared integrated photonics on silicon: a perspective. Nanophotonics, 7, 393-420(2018).
[9] V. Sorianello, M. Midrio, G. Contestabile, I. Asselberghs, J. Van Campenhout, C. Huyghebaert, I. Goykhman, A. K. Ott, A. C. Ferrari, M. Romagnoli. Graphene–silicon phase modulators with gigahertz bandwidth. Nat. Photonics, 12, 40-44(2018).
[10] I. Datta, S. H. Chae, G. R. Bhatt, M. A. Tadayon, B. Li, Y. Yu, C. Park, J. Park, L. Cao, D. N. Basov, J. Hone, M. Lipson. Low-loss composite photonic platform based on 2D semiconductor monolayers. Nat. Photonics, 14, 256-262(2020).
[11] R. Zhang, J. A. Ma. Simple bidirectional hybrid optical link for alternatively providing wired and wireless accesses based on an optical phase modulator. Photon. Netw. Commun., 34, 478-485(2017).
[12] W. Zhang, M. Ebert, B. Chen, J. D. Reynolds, X. Yan, H. Du, M. Banakar, D. T. Tran, K. Debnath, C. G. Littlejohns, S. Saito, D. J. Thomson. Integration of low loss vertical slot waveguides on SOI photonic platforms for high efficiency carrier accumulation modulators. Opt. Express, 28, 23143-23153(2020).
[13] F. Eltes, G. E. Villarreal-Garcia, D. Caimi, H. Siegwart, A. A. Gentile, A. Hart, P. Stark, G. D. Marshall, M. G. Thompson, J. Barreto, J. Fompeyrine, S. Abel. An integrated optical modulator operating at cryogenic temperatures. Nat. Mater., 19, 1164-1168(2020).
[14] J. E. Ortmann, F. Eltes, D. Caimi, N. Meier, A. A. Demkov, L. Czornomaz, J. Fompeyrine, S. Abel. Ultra-low-power tuning in hybrid barium titanate–silicon nitride electro-optic devices on silicon. ACS Photon., 6, 2677-2684(2019).
[15] N. Ahmed, S. Shi, A. J. Mercante, D. W. Prather. High-performance racetrack resonator in silicon nitride thin film lithium niobate hybrid platform. Opt. Express, 27, 30741-30751(2019).
[16] K. Alexander, J. P. George, J. Verbist, K. Neyts, B. Kuyken, D. V. Thourhout, J. Beeckman. Nanophotonic Pockels modulators on a silicon nitride platform. Nat. Commun., 9, 3444(2018).
[17] P. Zhang, H. Huang, Y. Jiang, X. Han, H. Xiao, A. Frigg, T. G. Nguyen, A. Boes, G. Ren, Y. Su, Y. Tian, A. Mitchell. High-speed electro-optic modulator based on silicon nitride loaded lithium niobate on an insulator platform. Opt. Lett., 46, 5986-5989(2021).
[18] C. Phare, Y. H. Daniel Lee, J. Cardenas, M. Lipson. Graphene electro-optic modulator with 30 GHz bandwidth. Nat. Photonics, 9, 511-514(2015).
[19] J. Wang, X. Zhang, Y. Chen, Y. Geng, Y. Du, X. Li. Design of a graphene-based silicon nitride multimode waveguide-integrated electro-optic modulator. Opt. Commun., 481, 126531(2021).
[20] C. Qiu, Y. Yang, C. Li, Y. Wang, K. Wu, J. Chen. All-optical control of light on a graphene-on-silicon nitride chip using thermo-optic effect. Sci. Rep., 7, 17046(2017).
[21] Z. Ruan, L. Pei, J. Wang, J. Zheng, J. Wang, J. Li, T. Ning, Q. Zhao, Y. Xi. All-optical graphene oxide modulator based on phase-shifted FBG. J. Lightwave Technol., 39, 5516-5522(2021).
[22] Y. Meng, S. Ye, Y. Shen, Q. Xiao, X. Fu, R. Lu, Y. Liu, M. Gong. Waveguide engineering of graphene optoelectronics—modulators and polarizers. IEEE Photon. J., 10, 6600217(2018).
[23] K. F. Mak, C. Lee, J. Hone, J. Shan, T. F. Heinz. Atomically thin MoS2: a new direct-gap semiconductor. Phys. Rev. Lett., 105, 136805(2010).
[24] T. Chu, H. Ilatikhameneh, G. Klimeck, R. Rahman, Z. Chen. Electrically tunable bandgaps in bilayer MoS2. Nano Lett., 15, 8000-8007(2015).
[25] K. Mak, K. He, C. Lee, J. Hone, T. F. Heinz, J. Shan. Tightly bound trions in monolayer MoS2. Nat. Mater., 12, 207-211(2013).
[26] N. Peimyoo, T. Deilmann, F. Withers, J. Escolar, D. Nutting, T. Taniguchi, K. Watanabe, A. Taghizadeh, M. F. Craciun, K. S. Thygesen, S. Russo. Electrical tuning of optically active interlayer excitons in bilayer MoS2. Nat. Nanotechnol., 16, 888-893(2021).
[27] W. Zhao, S. Bi, C. Zhang, P. D. Rack, G. Feng. Adding solvent into ionic liquid-gated transistor: the anatomy of enhanced gating performance. ACS Appl. Mater. Interfaces, 11, 13822-13830(2019).
[28] Y. Zhang, J. Ye, Y. Matsuhashi, Y. Iwasa. Ambipolar MoS2 thin flake transistors. Nano Lett., 12, 1136-1140(2012).
[29] T. H. Tsai, A. K. Sahoo, H. K. Syu, Y. C. Wu, M. Y. Tsai, M. D. Siao, Y. C. Yang, Y. F. Lin, R. S. Liu, P. W. Chiu. WS2/WSe2 nanodot composite photodetectors for fast and sensitive light detection. ACS Appl. Electron. Mater., 3, 4291-4299(2021).
[30] Y. Sun, R. Hu, C. An, X. Ma, J. Zhang, J. Liu. Visible to near-infrared photodetector based on SnSe2/WSe2 heterojunction with potential application in artificial visual neuron. Nanotechnology, 32, 475206(2021).
[31] G. Wei, T. K. Stanev, D. A. Czaplewski, I. W. Jung, N. P. Stern. Silicon-nitride photonic circuits interfaced with monolayer MoS2. Appl. Phys. Lett., 107, 091112(2015).
[32] Y. Dai, Y. Wang, S. Das, H. Xue, X. Bai, E. Hulkko, Z. Sun. Electrical control of interband resonant nonlinear optics in monolayer MoS2. ACS Nano, 14, 8442-8448(2020).
[33] E. J. G. Santos, E. Kaxiras. Electrically driven tuning of the dielectric constant in MoS2 layers. ACS Nano, 7, 10741-10746(2013).
[34] S. Wang, H. Yu, H. Zhang, A. Wang, M. Zhao, Y. Chen, L. Mei, J. Wang. Broadband few-layer MoS2 saturable absorbers. Adv. Mater., 26, 3538-3544(2014).
[35] Y. Zhou, L. Tao, Z. Chen, H. Lai, W. Xie, J. Xu. Defect etching of phase-transition-assisted CVD-grown 2H-MoTe2. Small, 17, 2102146(2021).
[36] B. Radisavljevic, A. Radenovic, J. Brivio, V. Giacometti, A. Kis. Single-layer MoS2 transistors. Nat. Nanotechnol., 6, 147-150(2011).
[37] Y. Rho, J. Pei, L. Wang, Z. Su, M. Eliceiri, C. P. Grigoropoulos. Site-selective atomic layer precision thinning of MoS2 via laser-assisted anisotropic chemical etching. ACS Appl. Mater. Interfaces, 11, 39385-39393(2019).
[38] X. Ji, F. Barbosa, S. P. Roberts, A. Dutt, J. Cardenas, Y. Okawachi, A. Bryant, A. L. Gaeta, M. Lipson. Ultra-low-loss on-chip resonators with sub-milliwatt parametric oscillation threshold. Optica, 4, 619-624(2017).
[39] M. Zhang, R. C. Howe, R. I. Woodward, E. J. Kelleher, F. Torrisi, G. Hu, T. Hasan. Solution processed MoS2-PVA composite for sub-bandgap mode-locking of a wideband tunable ultrafast Er:fiber laser. Nano Res., 8, 1522-1534(2015).
[40] K. Wu, X. Zhang, J. Wang, J. Chen. 463-MHz fundamental mode-locked fiber laser based on few-layer MoS2 saturable absorber. Opt. Lett., 40, 1374-1377(2015).
[41] A. Pezeshki, S. H. H. Shokouh, T. Nazari, K. Oh, S. Im. Electric and photovoltaic behavior of a few-layer α-MoTe2/MoS2 dichalcogenide heterojunction. Adv. Mater., 28, 3216-3222(2016).
[42] S. Rathi, I. Lee, D. Lim, J. Wang, Y. Ochiai, N. Aoki, K. Watanabe, T. Taniguchi, G.-H. Lee, Y.-J. Yu, P. Kim, G.-H. Kim. Tunable electrical and optical characteristics in monolayer graphene and few-layer MoS2 heterostructure devices. Nano Lett., 15, 5017-5024(2015).
[43] D. Braga, I. G. Lezama, H. Berger, A. F. Morpurgo. Quantitative determination of the band gap of WS2 with ambipolar ionic liquid-gated transistors. Nano Lett., 12, 5218-5223(2012).
[44] A. Kuc, N. Zibouche, T. Heine. Influence of quantum confinement on the electronic structure of the transition metal sulfide TS2. Phys. Rev. B., 83, 245213(2011).
[45] Y. Ding, X. Zhu, S. Xiao, H. Hu, L. H. Frandsen, N. A. Mortensen, K. Yvind. Effective electro-optical modulation with high extinction ratio by a graphene–silicon microring resonator. Nano Lett., 15, 4393-4400(2015).
[46] M. He, M. Xu, Y. Ren, J. Jian, Z. Ruan, Y. Xu, S. Gao, S. Sun, X. Wen, L. Zhou, L. Liu, C. Guo, H. Chen, S. Yu, L. Liu, X. Cai. High-performance hybrid silicon and lithium niobate Mach–Zehnder modulators for 100 Gbit s−1 and beyond. Nat. Photonics, 13, 359-364(2019).
[47] M. Li, L. Wang, X. Li, X. Xiao, S. Yu. Silicon intensity Mach–Zehnder modulator for single lane 100 Gb/s applications. Photon. Res., 6, 109-116(2018).
[48] R. Amin, R. Maiti, Y. Gui, C. Suer, M. Miscuglio, E. Heidari, R. T. Chen, H. Dalir, V. J. Sorger. Sub-wavelength GHz-fast broadband ITO Mach–Zehnder modulator on silicon photonics. Optica, 7, 333-335(2020).
[49] W. Jin, R. G. Polcawich, P. A. Morton, J. E. Bowers. Piezoelectrically tuned silicon nitride ring resonator. Opt. Express, 26, 3174-3187(2018).
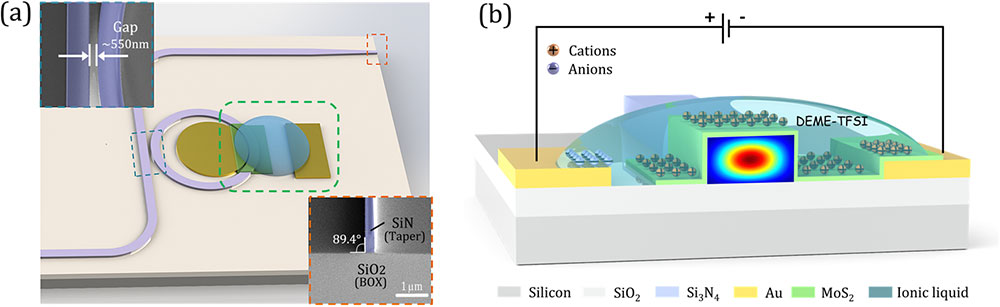
Set citation alerts for the article
Please enter your email address