
- Journal of Semiconductors
- Vol. 43, Issue 6, 062802 (2022)
Abstract
1. Introduction
In recent years, gallium oxide (Ga2O3) has been quickly emerging as a viable semiconductor with great application potential in several kinds of important functional devices, including power electronics, solar blind UV photodetectors, and ultrasensitive sensors due to its super wide bandgap (WBG) and other outstanding properties[
In this study, we attempt to present an investigation on the above-mentioned subjects. We first elucidate the distinct dispersion of the experimental sub-bandgap refractive indexes with a new model proposed by two (Bao and Xu) of the present authors for semiconducting and insulating WBG materials[
2. The results and discussion
Fig. 1 shows the calculated (solid line) and the experimental (solid squares) refractive indexes of β-Ga2O3 thin films as a function of photon energy. The experimental data were reported by Rebien et al.[
Figure 1.(Color online) The experimental (solid squares) and calculated (solid line) refractive indexes of
Fig. 2 shows measured sub-bandgap transmission spectra (solid squares) of the Si-doped β-Ga2O3 thin films grown on c-plane sapphire substrates by Hu et al.[
Figure 2.(Color online) The measured transmission spectra (solid squares) and corresponding fitting curves (red solid lines) of the
where n0 is a background refractive index, i.e., static refractive index, the transmission spectrum of thin film due to the thin-film interference effect may be formulated as[
where
The solid lines in Fig. 2 represent the simulation curves with Eq. (2). Good agreement between theory and experiment is achieved, which leads to the determination of several key parameters such as the film thickness and effective optical bandgap. The determined thin film thickness was ~500 nm. It is regrettable that there were no experimental thickness data of the thin films reported in Ref. [4]. The obtained effective optical bandgap values are tabulated in Table 1. The experimental flow rates of SiH4 are listed in Table 1 too. From Table 1, the effective optical bandgap of intentionally undoped β-Ga2O3 thin film was 4.952 eV. Available bandgap values of β-Ga2O3 crystal are quite scattered, i.e., 4.7−5.04 eV, probably due to different theoretical approaches, doping concentrations, and crystal orientations[
It is well known that ionized dopants in solid can produce electric fields around them inside solid. Such ionized-dopant-induced electric fields may result in some significant effects, such as their substantial impact on the band-edge absorption of insulating solids[
Figure 3.(Color online) Measured (open circles) and calculated (solid lines) sub-bandgap absorption coefficients of
where
where
3. Conclusions
In conclusion, the dispersion of the refractive index of β-Ga2O3 thin film in the sub-bandgap energy region was elucidated. Based on the peculiar dispersion of sub-bandgap refractive index, the variable-period oscillation patterns in the measured sub-bandgap transmission spectra of Si-doped β-Ga2O3 thin films with different doping concentrations were quantitatively interpreted. It is found that the effective optical bandgap of the films decreases with increasing the Si doping concentration. Under the frame of Franz–Keldysh mechanism due to the electric fields, the sub-bandgap absorption coefficients of β-Ga2O3 were calculated. It is shown that the electric fields induced by ionized Si impurities should be responsible for the sub-bandgap absorption in β-Ga2O3 thin films. These new findings not only deepen the existing understanding of the sub-bandgap refractive indexes and optical properties of β-Ga2O3, but also may promote the further device applications of this unique WBG oxide semiconductor.
Acknowledgements
This study was financially supported by the National Natural Science Foundation of China (No. 12074324) and the Shenzhen Municipal Science and Technology Innovation Council (No. JCJY20180508163404043).
References
[1] S J Pearton, J Yang, IV P H Cary et al. A review of Ga2O3 materials, processing, and device. Appl Phys Rev, 5, 011301(2018).
[2] H F Mohamed, C Xia, Q Sai et al. Growth and fundamentals of bulk
[3] F Zhang, M Arita, X Wang et al. Toward controlling the carrier density of Si doped Ga2O3 films by pulsed laser deposition. Appl Phys Lett, 109, 102105(2016).
[4] D Hu, Y Wang, S Zhuang et al. Surface morphology evolution and optoelectronic properties of heteroepitaxial Si-doped
[5] S J Pearton, F Ren, M Tadjer et al. Perspective: Ga2O3 for ultra-high power rectifiers and MOSFETS. J Appl Phys, 124, 220901(2018).
[6] J Yang, S Ahn, F Ren et al. High breakdown voltage (−201)
[7] B Wang, M Xiao, X Yan et al. High-voltage vertical Ga2O3 power rectifiers operational at high temperatures up to 600 K. Appl Phys Lett, 115, 263503(2019).
[8] K D Chabak, J P McCandless, N A Moser et al. Recessed-gate enhancement-mode
[9] A J Green, K D Chabak, M Baldini et al.
[10] N Moser, J McCandless, A Crespo et al. Ge-doped
[11] S Oh, C K Kim, J Kim. High responsivity
[12] Y C Chen, Y J Lu, Q Liu et al. Ga2O3 photodetector arrays for solar-blind imaging. J Mater Chem C, 7, 2557(2019).
[13] J Xu, W Zheng, F Huang. Gallium oxide solar-blind ultraviolet photodetectors: a review. J Mater Chem C, 7, 8753(2019).
[14] Z Liu, X Wang, Y Liu et al. A high-performance ultraviolet solar-blind photodetector based on a
[15] L Zhang, X Xiu, Y Li et al. Solar-blind ultraviolet photodetector based on vertically aligned single-crystalline
[16] C Xie, X Lu, Y Liang et al. Patterned growth of
[17] M Rebien, W Henrion, M Hong et al. Optical properties of gallium oxide thin films. Appl Phys Lett, 81, 250(2002).
[18] Y Bao, S Xu. Variable-period oscillations in optical spectra in sub-bandgap long wavelength region: Signatures of new dispersion of refractive index. J Phys D, 54, 155102(2021).
[19] I Bhaumik, R Bhatt, S Ganesamoorthy et al. Temperature-dependent index of refraction of monoclinic Ga2O3 single crystal. Appl Opt, 50, 6006(2011).
[20] N Ueda, H Hosono, R Waseda et al. Anisotropy of electrical and optical properties in
[21] M Hilfiker, U Kilic, A Mock et al. Dielectric function tensor (1.5 eV to 9.0 eV), anisotropy, and band to band transitions of monoclinic
[22] J Furthmüller, F Bechstedt. Quasiparticle bands and spectra of Ga2O3 polymorphs. Phys Rev B, 93, 115204(2016).
[23] J Yan, C Qu. Electronic structure and optical properties of F-doped-Ga2O3 from first principles calculations. J Semicond, 37, 042002(2016).
[24] A Mock, R Korlacki, C Briley et al. Band-to-band transitions, selection rules, effective mass, and excitonic contributions in monoclinic
[25] Z Galazka.
[26] D Redfield. Effect of defect fields on the optical absorption edge. Phys Rev, 130, 916(1963).
[27] K Tharmalingam. Optical absorption in the presence of a uniform field. Phys Rev, 130, 2204(1963).
[28] Y Bao, S Xu. Dopant-induced electric fields and their influence on the band-edge absorption of GaN. ACS Omega, 4, 15401(2019).
[29] S Rafique, L Han, S Mou et al. Temperature and doping concentration dependence of the energy band gap in
[30] R Subrina, L Han, H Zhao. Synthesis of wide bandgap Ga2O3 (
[31] J Zhang, J Shi, D C Qi et al. Recent progress on the electronic structure, defect, and doping properties of Ga2O3. APL Mater, 8, 020906(2020).
[32] H Peelaers, C G Van de Walle. Sub-band-gap absorption in Ga2O3. Appl Phys Lett, 111, 182104(2017).
[33] F Bechstedt, J Furthmüller. Influence of screening dynamics on excitons in Ga2O3 polymorphs. Appl Phys Lett, 114, 122101(2019).
[34] R Guo, J Su, H Yuan et al. Surface functionalization modulates the structural and optoelectronic properties of two-dimensional Ga2O3. Mater Today Phys, 12, 100192(2020).
[35] S L Shi, S J Xu. Determination of effective mass of heavy hole from phonon-assisted excitonic luminescence spectra in ZnO. J Appl Phys, 109, 053510(2011).
[36] X Wang, D Yu, S Xu. Determination of absorption coefficients and Urbach tail depth of ZnO below the bandgap with two-photon photoluminescence. Opt Express, 28, 13817(2020).
[37] H G Ye, Z C Su, F Tang et al. Role of free electrons in phosphorescence in n-type wide bandgap semiconductors. Phys Chem Chem Phys, 19, 30332(2017).
[38] H Ye, Z Su, F Tang et al. Probing defects in ZnO by persistent phosphorescence. Opto-Electron Adv, 1, 180011(2018).
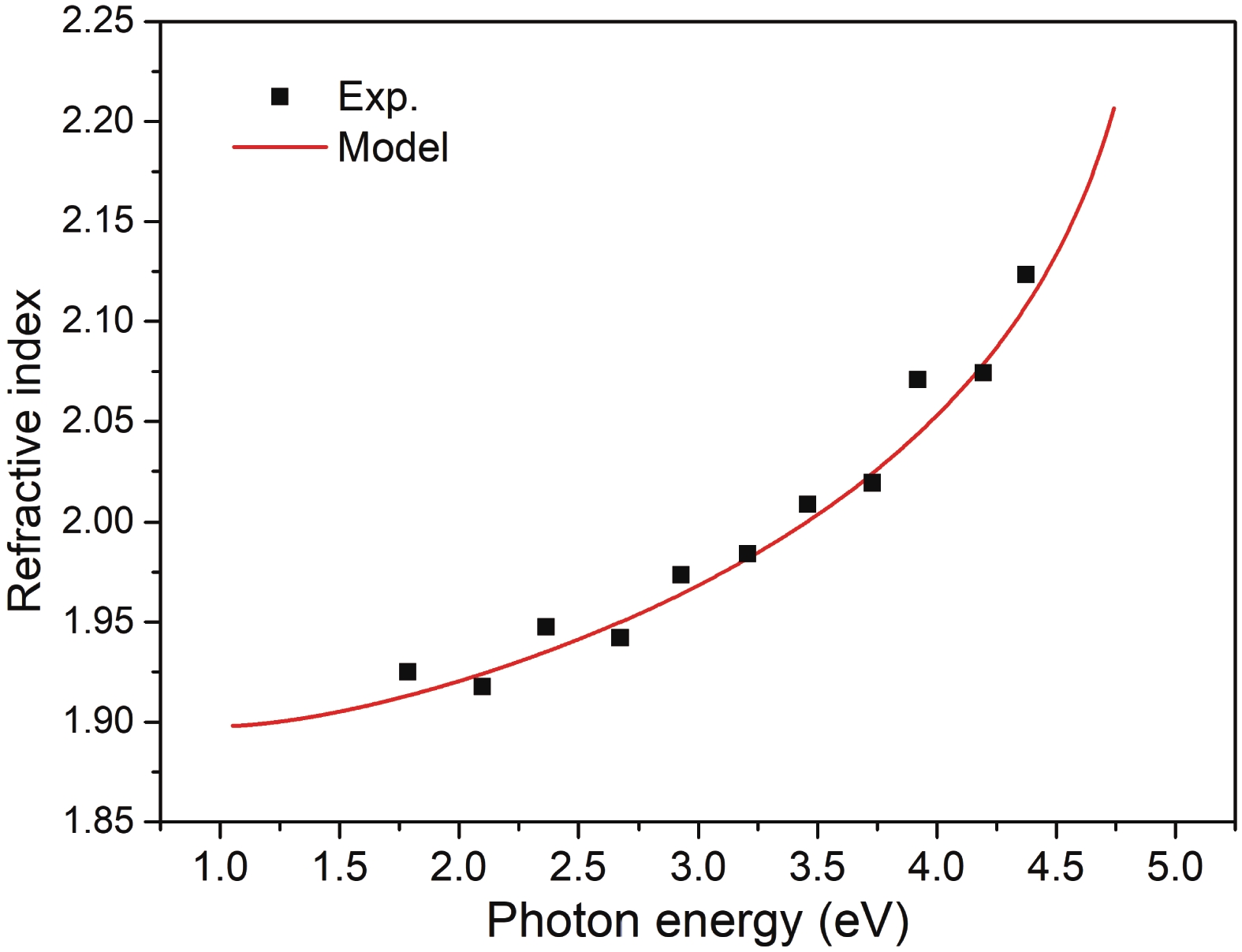
Set citation alerts for the article
Please enter your email address