
- Photonics Research
- Vol. 10, Issue 6, 1440 (2022)
Abstract
1. INTRODUCTION
Near infrared semiconductor nanolasers are of great significance for integrated optoelectronic chips [1–3]. An efficient gain medium is one of the key components of near infrared nanolasers [4–6]. The traditional gain media of near infrared lasers are made of inorganic semiconductors, but their quantum efficiencies are low and the growths require critical conditions [3]. Perovskites have attracted considerable interests and have been considered as leading-candidate gain media for next generation on-chip optical sources, thanks to their outstanding photophysical properties as well as low cost and promise for electrically driven lasing [7–10]. Among various perovskite materials, organic–inorganic hybrid materials, with
In recent years, organic–inorganic hybrid perovskite lasers have achieved rapid progress. Zhang
However, organic and inorganic hybrid perovskites suffer from instability under operating conditions. It was reported that the temperature of a distributed feedback
Sign up for Photonics Research TOC. Get the latest issue of Photonics Research delivered right to you!Sign up now
On the one hand, promoting the operating stability of lasers is one of the constant tasks of laser technology [28]. Although room-temperature continuous wave perovskite lasers have been reported [17], one of the major hurdles towards electrically pumped lasers is resistive heating under current injection [7]. On the other hand, improving the thermal stability is of critical importance for achieving electrically pumped perovskite lasers. Until now, great efforts have been made to improve the stability of organic–inorganic perovskites while maintaining their outstanding photophysical properties [29–31]. Working at cryogenic temperatures to keep perovskites below thermal degradation temperature is helpful to promote the stability of perovskite lasers. For example, CW amplified spontaneous emission (ASE) in a phase-stable perovskite has been demonstrated at temperatures up to 120 K [32]. However, room-temperature operating lasers are preferred in most applications [28]. The encapsulation strategy has been resorted to improve the perovskite lasing stability. For example, a thin poly-methyl-methacrylate (PMMA) encapsulation layer was applied in an
Understanding the degradation mechanisms is of significant importance for improving the operating stability of perovskite lasers.
In this work, by continuously monitoring the emission properties of an
2. RESULTS AND DISCUSSION
The
The degradation evolution of an
Figure 1.(a) Microscopic image of an
The emission spectrum evolutions of the laser operating at a pump intensity of
From the microscopic image of the initial
The observed phenomenon of
In contrast to fully converting
Figure 2.(a) Microscopic image of
The influence of excess
We also measured the TRPL as shown in Fig. 2(i). At a pump intensity of
The operational stability of the
Figure 3.(a) Microscopic images of a
Next, we optimized the operational stability of a
To suppress the surface-initiated degradation of perovskite nanoplatelets, we employed a thin DBP film as the encapsulation layer on a newly synthesized
Figure 4.(a) Schematic diagram of passivating the surface of
The lasing performance of the DBP encapsulated
We then performed the operational stability test of the obtained stable
The average operation times of unpassivated (sample A),
3. CONCLUSION
In conclusion, a high stability
Acknowledgment
Acknowledgment. YC also acknowledges support from Key Research and Development, Henry Fok Education Foundation Young Teachers Fund, and Platform and Base Special Project of Shanxi Province. HZ also acknowledges support from the Natural Science Foundation of Guangdong Province, Shenzhen Nanshan District Pilotage Team Program, and the Science and Technology Innovation Commission of Shenzhen.
APPENDIX A: EXPERIMENTAL METHODS
0.002 g DBP (99%, Han Feng) was first fully dissolved in 1 mL chlorobenzene (Sigma). After filtration, 20 μL DBP solution was spin-coated on the surface of the perovskite nanoplatelets at 4500 r/min for 30 s in a
The optical images of
We carried out optically pumped lasing measurements on a home-built microscope setup. The 343 nm excitation pulses were generated by frequency tripling the 1028 nm output (with a BBO crystal) from a light conversion carbide femtosecond laser (290 fs, 6 kHz, 1028 nm). The pumping source was focused onto samples via an uncoated convex lens (focal length, 20 cm; transmittance, 80%). To ensure uniform energy injection, the laser spot diameter was focused to
The emission intensity from a single nanoplatelet was monitored using an ideaoptics PG2000-Pro spectrometer with a wavelength resolution (FWHM) of 0.3 nm in the range of 700–900 nm. Since the spectrometer does not require cooling liquid, it can work stably for longer. For the spectral range of 200–1100 nm, the ideaoptics PG2000 spectrometer with a wavelength resolution (FWHM) of 1.3 nm was used.
APPENDIX B: CHARACTERIZATION OF UNPASSIVATED MAPbI3 LASERS
Laser performance of an
Figure 5.(a) Laser output intensity as a function of pump intensity. (b) Evolution of emission spectra obtained at different pump intensities. (c) TRPL spectra of a perovskite nanoplatelet without passivation operating at spontaneous emission (
Figure 6.Lasing stability data of two other unpassivated
Figure 7.Emission spectra of an unpassivated
Figure 8.(a) AFM image of the edge of the unpassivated
Figure 9.XRD patterns of
APPENDIX C: POPULATION INVERSION RELATED LASER OUTPUT
A light wave traveling through a nanoplatelet as shown in Fig.
Figure 10.Schematic diagram of the light path in an
The output power from a laser is
Since
Therefore, more
APPENDIX D: SIMULATION OF TRANSIENT THERMAL RESPONSE OF AN MAPbI3 NANOPLATELET
A 3D heat transfer model is solved by the finite difference method to determine the time-dependent temperature distribution in the perovskite nanoplatelets. The hexagonal
Parameters of the Materials Used for Transient Thermal Response Simulation
Type | Mica | |
---|---|---|
Absorption coefficient [ | ||
Thermal conductivity [ | 0.5 [ | 0.75 [ |
Density [ | 3947 [ | 2900 [ |
Heat capacity [ | 241.9 [ | 880 [ |
Figure 11.(a) Schematic diagram of an
Figure
APPENDIX E: CHARACTERIZATION OF PbI2 PASSIVATED MAPbI3 LASERS
The thickness of the
Figure 12.(a) AFM image of the edge of
Figure 13.Lasing stability data of another two
APPENDIX F: CHARACTERIZATION OF DUAL PASSIVATED MAPbI3 LASERS
Image of DBP passivated and unpassivated
Figure 14.(a) Image of the
Figure 15.Emission spectra of an unpassivated
Figure 16.Microscopic images of an
Figure 17.Microscopic images of the
Figure 18.Lasing stability data of another two dual passivation processed
APPENDIX G: COMPARISON OF OPERATIONAL STABILITY OF DIFFERENT MAPbI3 LASERS
The average operation time of unpassivated (sample A),
Figure 19.Average operation time of unpassivated (sample A),
References
[1] H. Zhu, Y. Fu, F. Meng, X. Wu, Z. Gong, Q. Ding, M. V. Gustafsson, M. T. Trinh, S. Jin, X. Y. Zhu. Lead halide perovskite nanowire lasers with low lasing thresholds and high quality factors. Nat. Mater., 14, 636-642(2015).
[2] B. R. Sutherland, S. Hoogland, M. M. Adachi, C. T. O. Wong, E. H. Sargent. Conformal organohalide perovskites enable lasing on spherical resonators. ACS Nano, 8, 10947-10952(2014).
[3] Q. Zhang, S. T. Ha, X. Liu, T. C. Sum, Q. Xiong. Room-temperature near-infrared high-
[4] M. T. Hill, M. C. Gather. Advances in small lasers. Nat. Photonics, 8, 908-918(2014).
[5] Q. Wei, X. Li, C. Liang, Z. Zhang, J. Guo, G. Hong, G. Xing, W. Huang. Recent progress in metal halide perovskite micro- and nanolasers. Adv. Opt. Mater., 7, 1900080(2019).
[6] K. Wang, S. Wang, S. Xiao, Q. Song. Recent advances in perovskite micro- and nanolasers. Adv. Opt. Mater., 6, 1800278(2018).
[7] B. R. Sutherland, E. H. Sargent. Perovskite photonic sources. Nat. Photonics, 10, 295-302(2016).
[8] Y. Zhang, C.-K. Lim, Z. Dai, G. Yu, J. W. Haus, H. Zhang, P. N. Prasad. Photonics and optoelectronics using nano-structured hybrid perovskite media and their optical cavities. Phys. Rep., 795, 1-51(2019).
[9] A. P. Schlaus, M. S. Spencer, K. Miyata, F. Liu, X. Wang, I. Datta, M. Lipson, A. Pan, X. Y. Zhu. How lasing happens in CsPbBr3 perovskite nanowires. Nat. Commun., 10, 265(2019).
[10] Y. Liu, J. Cui, K. Du, H. Tian, Z. He, Q. Zhou, Z. Yang, Y. Deng, D. Chen, X. Zuo, Y. Ren, L. Wang, H. Zhu, B. Zhao, D. Di, J. Wang, R. H. Friend, Y. Jin. Efficient blue light-emitting diodes based on quantum-confined bromide perovskite nanostructures. Nat. Photonics, 13, 760-764(2019).
[11] M. Green, E. Dunlop, J. Hohl-Ebinger, M. Yoshita, N. Kopidakis, X. Hao. Solar cell efficiency tables (version 57). Prog. Photovolt. Res. Appl., 29, 3-15(2021).
[12] Y. Chen, X. Zuo, Y. He, F. Qian, S. Zuo, Y. Zhang, L. Liang, Z. Chen, K. Zhao, Z. Liu, J. Gou, S. Liu. Dual passivation of perovskite and SnO2 for high-efficiency MAPbI3 perovskite solar cells. Adv. Sci., 8, 2001466(2021).
[13] S. A. Veldhuis, P. P. Boix, N. Yantara, M. Li, T. C. Sum, N. Mathews, S. G. Mhaisalkar. Perovskite materials for light-emitting diodes and lasers. Adv. Mater., 28, 6804-6834(2016).
[14] G. Li, R. Gao, Y. Han, A. Zhai, Y. Liu, Y. Tian, B. Tian, Y. Hao, S. Liu, Y. Wu, Y. Cui. High detectivity photodetectors based on perovskite nanowires with suppressed surface defects. Photon. Res., 8, 1862-1874(2020).
[15] J. S. Manser, J. A. Christians, P. V. Kamat. Intriguing optoelectronic properties of metal halide perovskites. Chem. Rev., 116, 12956-13008(2016).
[16] Y. Jia, R. A. Kerner, A. J. Grede, B. P. Rand, N. C. Giebink. Continuous-wave lasing in an organic–inorganic lead halide perovskite semiconductor. Nat. Photonics, 11, 784-788(2017).
[17] C. Qin, A. S. D. Sandanayaka, C. Zhao, T. Matsushima, D. Zhang, T. Fujihara, C. Adachi. Stable room-temperature continuous-wave lasing in quasi-2D perovskite films. Nature, 585, 53-57(2020).
[18] S. W. Eaton, M. Lai, N. A. Gibson, A. B. Wong, L. Dou, J. Ma, L.-W. Wang, S. R. Leone, P. Yang. Lasing in robust cesium lead halide perovskite nanowires. Proc. Natl. Acad. Sci. USA, 113, 1993-1998(2016).
[19] B. Tang, H. Dong, L. Sun, W. Zheng, Q. Wang, F. Sun, X. Jiang, A. Pan, L. Zhang. Single-mode lasers based on cesium lead halide perovskite submicron spheres. ACS Nano, 11, 10681-10688(2017).
[20] C. Zhao, W. Tian, J. Liu, Q. Sun, J. Luo, H. Yuan, B. Gai, J. Tang, J. Guo, S. Jin. Stable two-photon pumped amplified spontaneous emission from millimeter-sized CsPbBr3 single crystals. J. Phys. Chem. Lett., 10, 2357-2362(2019).
[21] M.-G. Ju, M. Chen, Y. Zhou, J. Dai, L. Ma, N. P. Padture, X. C. Zeng. Toward eco-friendly and stable perovskite materials for photovoltaics. Joule, 2, 1231-1241(2018).
[22] Y. Yan, T. Pullerits, K. Zheng, Z. Liang. Advancing tin halide perovskites: strategies toward the ASnX3 paradigm for efficient and durable optoelectronics. ACS Energy Lett., 5, 2052-2086(2020).
[23] J. Luo, X. Wang, S. Li, J. Liu, Y. Guo, G. Niu, L. Yao, Y. Fu, L. Gao, Q. Dong, C. Zhao, M. Leng, F. Ma, W. Liang, L. Wang, S. Jin, J. Han, L. Zhang, J. Etheridge, J. Wang, Y. Yan, E. H. Sargent, J. Tang. Efficient and stable emission of warm-white light from lead-free halide double perovskites. Nature, 563, 541-545(2018).
[24] Y. Jia, R. A. Kerner, A. J. Grede, B. P. Rand, N. C. Giebink. Factors that limit continuous-wave lasing in hybrid perovskite semiconductors. Adv. Opt. Mater., 8, 1901514(2020).
[25] Z. Fan, H. Xiao, Y. Wang, Z. Zhao, Z. Lin, H.-C. Cheng, S.-J. Lee, G. Wang, Z. Feng, W. A. Goddard, Y. Huang, X. Duan. Layer-by-layer degradation of methylammonium lead tri-iodide perovskite microplates. Joule, 1, 548-562(2017).
[26] F. Mathies, P. Brenner, G. Hernandez-Sosa, I. A. Howard, U. W. Paetzold, U. Lemmer. Inkjet-printed perovskite distributed feedback lasers. Opt. Express, 26, A144-A152(2018).
[27] X. Li, K. Wang, M. Chen, S. Wang, Y. Fan, T. Liang, Q. Song, G. Xing, Z. Tang. Stable whispering gallery mode lasing from solution-processed formamidinium lead bromide perovskite microdisks. Adv. Opt. Mater., 8, 2000030(2020).
[28] Q. Zhang, Q. Shang, R. Su, T. T. H. Do, Q. Xiong. Halide perovskite semiconductor lasers: materials, cavity design, and low threshold. Nano Lett., 21, 1903-1914(2021).
[29] G. Li, K. Chen, Y. Cui, Y. Zhang, Y. Tian, B. Tian, Y. Hao, Y. Wu, H. Zhang. Stability of perovskite light sources: status and challenges. Adv. Opt. Mater., 8, 1902012(2020).
[30] T. Leijtens, G. E. Eperon, N. K. Noel, S. N. Habisreutinger, A. Petrozza, H. J. Snaith. Stability of metal halide perovskite solar cells. Adv. Energy Mater., 5, 1500963(2015).
[31] Q. Fu, X. Tang, B. Huang, T. Hu, L. Tan, L. Chen, Y. Chen. Recent progress on the long-term stability of perovskite solar cells. Adv. Sci., 5, 1700387(2018).
[32] P. Brenner, O. Bar-On, M. Jakoby, I. Allegro, B. S. Richards, U. W. Paetzold, I. A. Howard, J. Scheuer, U. Lemmer. Continuous wave amplified spontaneous emission in phase-stable lead halide perovskites. Nat. Commun., 10, 988(2019).
[33] S. Chen, K. Roh, J. Lee, W. K. Chong, Y. Lu, N. Mathews, T. C. Sum, A. Nurmikko. A photonic crystal laser from solution based organo-lead iodide perovskite thin films. ACS Nano, 10, 3959-3967(2016).
[34] G. L. Whitworth, J. R. Harwell, D. N. Miller, G. J. Hedley, W. Zhang, H. J. Snaith, G. A. Turnbull, I. D. Samuel. Nanoimprinted distributed feedback lasers of solution processed hybrid perovskites. Opt. Express, 24, 23677-23684(2016).
[35] J. Clark, G. Lanzani. Organic photonics for communications. Nat. Photonics, 4, 438-446(2010).
[36] K. Wang, G. Li, S. Wang, S. Liu, W. Sun, C. Huang, Y. Wang, Q. Song, S. Xiao. Dark-field sensors based on organometallic halide perovskite microlasers. Adv. Mater., 30, 1801481(2018).
[37] A. Khan. Laser diodes go green. Nat. Photonics, 3, 432-434(2009).
[38] K. Domanski, E. A. Alharbi, A. Hagfeldt, M. Grätzel, W. Tress. Systematic investigation of the impact of operation conditions on the degradation behaviour of perovskite solar cells. Nat. Energy, 3, 61-67(2018).
[39] N. Li, Y. Luo, Z. Chen, X. Niu, X. Zhang, J. Lu, R. Kumar, J. Jiang, H. Liu, X. Guo, B. Lai, G. Brocks, Q. Chen, S. Tao, D. P. Fenning, H. Zhou. Microscopic degradation in formamidinium-cesium lead iodide perovskite solar cells under operational stressors. Joule, 4, 1743-1758(2020).
[40] R. Guo, D. Han, W. Chen, L. Dai, K. Ji, Q. Xiong, S. Li, L. K. Reb, M. A. Scheel, S. Pratap, N. Li, S. Yin, T. Xiao, S. Liang, A. L. Oechsle, C. L. Weindl, M. Schwartzkopf, H. Ebert, P. Gao, K. Wang, M. Yuan, N. C. Greenham, S. D. Stranks, S. V. Roth, R. H. Friend, P. Müller-Buschbaum. Degradation mechanisms of perovskite solar cells under vacuum and one atmosphere of nitrogen. Nat. Energy, 6, 977-986(2021).
[41] D. Shi, V. Adinolfi, R. Comin, M. Yuan, E. Alarousu, A. Buin, Y. Chen, S. Hoogland, A. Rothenberger, K. Katsiev, Y. Losovyj, X. Zhang, P. A. Dowben, O. F. Mohammed, E. H. Sargent, O. M. Bakr. Low trap-state density and long carrier diffusion in organolead trihalide perovskite single crystals. Science, 347, 519-522(2015).
[42] G. Li, T. Che, X. Ji, S. Liu, Y. Hao, Y. Cui, S. Liu. Record-low-threshold lasers based on atomically smooth triangular nanoplatelet perovskite. Adv. Funct. Mater., 29, 1805553(2019).
[43] Y.-H. Deng. Perovskite decomposition and missing crystal planes in HRTEM. Nature, 594, E6-E7(2021).
[44] Y. Chen, Q. Meng, Y. Xiao, X. Zhang, J. Sun, C. B. Han, H. Gao, Y. Zhang, Y. Lu, H. Yan. Mechanism of PbI2
[45] S. T. Ha, X. Liu, Q. Zhang, D. Giovanni, T. C. Sum, Q. Xiong. Synthesis of organic–inorganic lead halide perovskite nanoplatelets: towards high-performance perovskite solar cells and optoelectronic devices. Adv. Opt. Mater., 2, 838-844(2014).
[46] F. Brivio, J. M. Frost, J. M. Skelton, A. J. Jackson, O. J. Weber, M. T. Weller, A. R. Goñi, A. M. A. Leguy, P. R. F. Barnes, A. Walsh. Lattice dynamics and vibrational spectra of the orthorhombic, tetragonal, and cubic phases of methylammonium lead iodide. Phys. Rev. B, 92, 144308(2015).
[47] Q. Chen, H. Zhou, T.-B. Song, S. Luo, Z. Hong, H.-S. Duan, L. Dou, Y. Liu, Y. Yang. Controllable self-induced passivation of hybrid lead iodide perovskites toward high performance solar cells. Nano Lett., 14, 4158-4163(2014).
[48] J. Li, S.-H. Wei, L.-W. Wang. Stability of the DX- center in GaAs quantum dots. Phys. Rev. Lett., 94, 185501(2005).
[49] J. Li, . Comparison between quantum confinement effects of quantum wires and dots. Chem. Mater., 16, 4012-4015(2004).
[50] S. Ding, S. Li, Q. Sun, Y. Wu, Y. Liu, Z. Li, Y. Cui, H. Wang, Y. Hao, Y. Wu. Enhanced performance of perovskite solar cells by the incorporation of the luminescent small molecule DBP: perovskite absorption spectrum modification and interface engineering. J. Mater. Chem. C, 7, 5686-5694(2019).
[51] T. Kirchhuebel, M. Gruenewald, F. Sojka, S. Kera, F. Bussolotti, T. Ueba, N. Ueno, G. Rouillé, R. Forker, T. Fritz. Self-assembly of tetraphenyldibenzoperiflanthene (DBP) films on Ag(111) in the monolayer regime. Langmuir, 32, 1981-1987(2016).
[52] Y. Wang, X. Li, J. Song, L. Xiao, H. Zeng, H. Sun. All-inorganic colloidal perovskite quantum dots: a new class of lasing materials with favorable characteristics. Adv. Mater., 27, 7101-7108(2015).
[53] W. G. Nagourney. Quantum Electronics for Atomic Physics(2010).
[54] Y. Wang, Y. Zhang, P. Zhang, W. Zhang. High intrinsic carrier mobility and photon absorption in the perovskite CH3NH3PbI3. Phys. Chem. Chem. Phys., 17, 11516-11520(2015).
[55] S. Wang, Q. Ai, T.-Q. Zou, C. Sun, M. Xie. Analysis of radiation effect on thermal conductivity measurement of semi-transparent materials based on transient plane source method. Appl. Therm. Eng., 177, 115457(2020).
[56] M. D. Birowosuto, D. Cortecchia, W. Drozdowski, K. Brylew, W. Lachmanski, A. Bruno, C. Soci. X-ray scintillation in lead halide perovskite crystals. Sci. Rep., 6, 37254(2016).
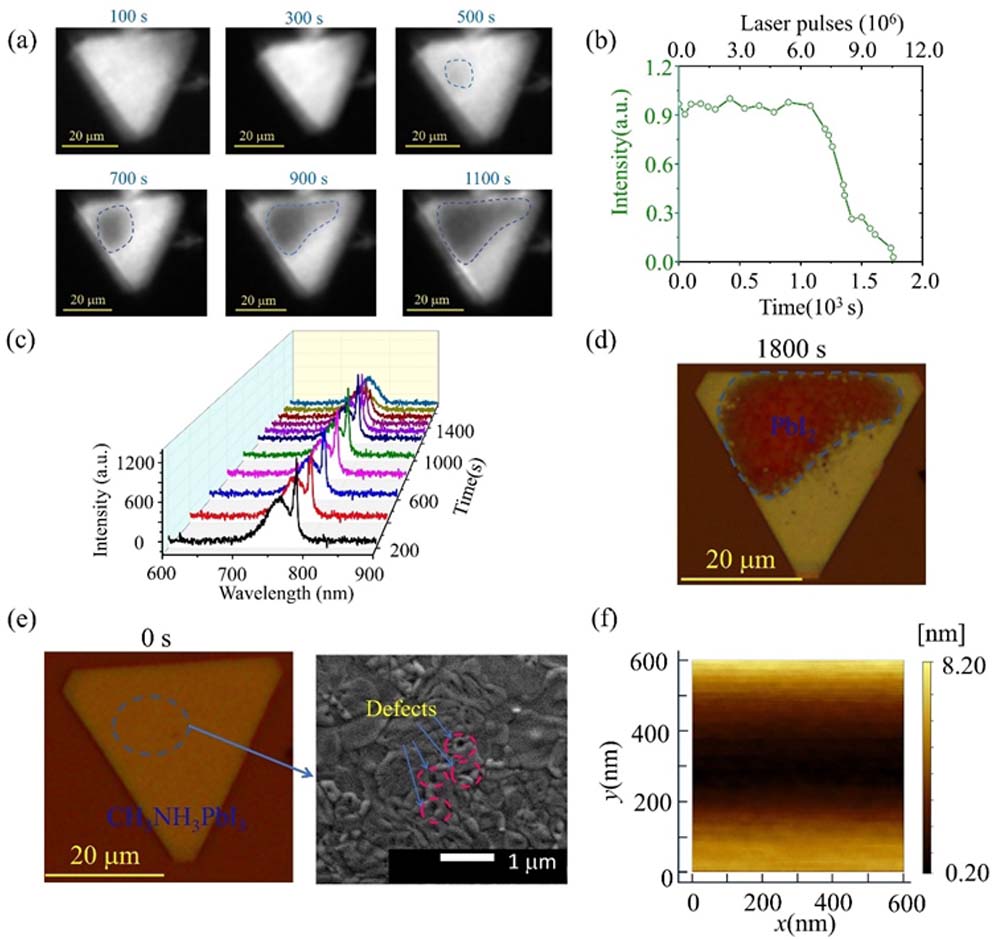
Set citation alerts for the article
Please enter your email address