Philip Menz, Haissam Hanafi, Daniel Leykam, Jörg Imbrock, Cornelia Denz, "Pseudospin-2 in photonic chiral borophene," Photonics Res. 11, 869 (2023)

Search by keywords or author
- Photonics Research
- Vol. 11, Issue 5, 869 (2023)
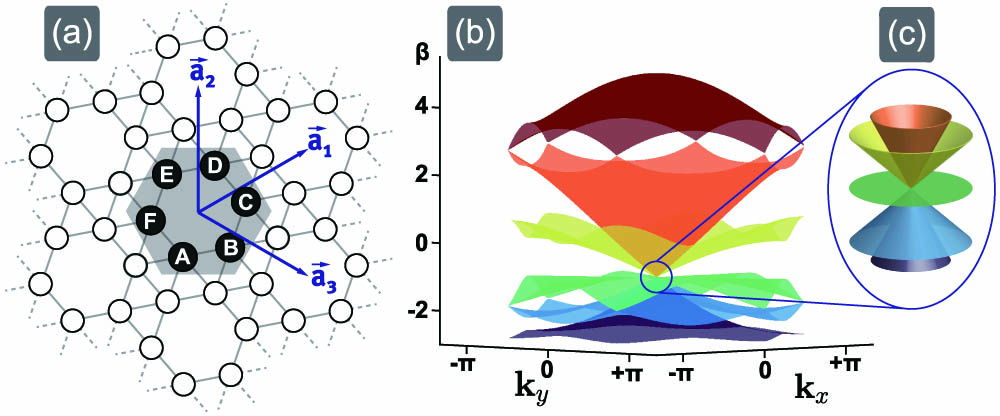
Fig. 1. Chiral borophene lattice and its band structure. (a) Schematic of the lattice with the unit cell in gray and lattice vectors a 1 , a 2 , and a 3 . The lattice sites are labeled from A to F. (b) Tight-binding band structure in the hexagonal Brillouin zone, calculated using d = t = 1 for nearest neighbors only. (c) Zoomed-in view of the linear dispersion close to the degenerate point showing an idealized pseudospin-2 conical intersection.
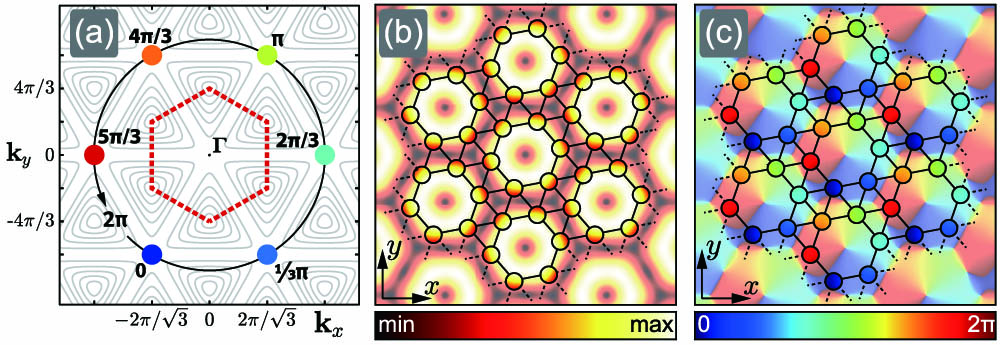
Fig. 2. Derivation of pseudospin eigenstate | ψ − 2 ⟩ . (a) Six plane waves in k -space at the Γ -points surrounding the first Brillouin zone (dashed red line) forming a hexagonal discrete vortex with l = + 1 . The shown isolines of the third (green) band help to identify the exact k -space excitation. (b), (c) Real space transverse amplitude and phase profile of the resulting discrete nondiffracting beam with overlaid chiral borophene lattice.
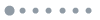
Fig. 3. Order of the five pseudospin states for m s increasing from m s = − 2 on the left to m s = + 2 on the right. The top row shows the discrete vorticity in k -space. The bottom row depicts the vortices of the corresponding discrete nondiffracting fields in real space, and therefore of the pseudospin eigenstates in the unit cell. The k -space and real space topological charges increase by unity from left to right. This is also reflected in the pseudospin value.
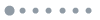
Fig. 4. Numerical simulation of conical diffraction and pseudospin-mediated vortex generation in photonic borophene. (a), (b) Amplitude and phase of the input light field given by the pseudospin state m s = − 2 multiplied by a Gaussian envelope. (c), (d) Output after propagation in the lattice. (e), (f) Same as (a), (b), but in Fourier space. (g), (h) Same as (c), (d), but in Fourier space. (i), (j) Zoom-in showing one of the spectral components. (k), (l) Ideal linear superposition of Laguerre–Gaussian modes LG 0,0 + LG 0 , − 1 + LG 0 , − 2 + LG 0 , − 3 + LG 0 , − 4 for comparison.
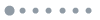
Fig. 5. Projection of the conical diffraction output field onto the five pseudospin eigenstates. Each hexagonal pixel represents one unit cell. (a), (b) Amplitude and phase of the projection onto | ψ − 2 ⟩ ; (c), (d) onto | ψ − 1 ⟩ ; (e), (f) onto | ψ 0 ⟩ ; (g), (h) onto | ψ + 1 ⟩ ; (i), (j) onto | ψ + 2 ⟩ .
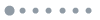
Fig. 6. Projection onto pseudospin eigenstates during propagation and conical diffraction of input state | ψ − 2 ⟩ . Percentages of different pseudospin eigenstates in the total field versus propagation distance in coupling lengths L c in the photonic lattice.
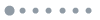
Fig. 7. Derivation of the pseudospin eigenstates | ψ − 1 ⟩ and | ψ 0 ⟩ . (a1) Six plane waves in k -space forming a hexagonal discrete vortex with l = + 2 . (a2), (a3) Real space transverse amplitude and phase profile of the resulting discrete nondiffracting beam with overlaid chiral borophene lattice for | ψ − 1 ⟩ . (b1) Same as in (a1) but with l = ± 3 . (b2), (b3) Same as in (a2) and (a3) but for | ψ 0 ⟩ .
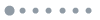
Fig. 8. Low-index mode. (a) Six plane waves in k -space without discrete vortex. (b), (c) Real space transverse amplitude and phase profile of the resulting discrete nondiffracting beam with overlaid chiral borophene lattice.
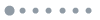
Fig. 9. Comparison of numerical simulations in tight-binding and continuous models. (a), (b) Amplitude and phase from solving the tight-binding coupled differential equations. (c), (d) Amplitude and phase from solving the continuous model via the split-step beam propagation method.
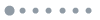
Fig. 10. Numerically simulated conical diffraction outputs of the remaining pseudospin eigenstates. (a), (b) Amplitude and phase after propagation of | ψ − 1 ⟩ in the chiral borophene lattice. (c), (d) Same as (a), (b), but for | ψ 0 ⟩ as input. (e), (f) Same as (a), (b), but for | ψ + 1 ⟩ as input. (g), (h) Same as (a), (b), but for | ψ + 2 ⟩ as input.
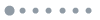
Fig. 11. Decompositions of the conical diffraction outputs of the remaining pseudospin eigenstates. The amplitudes for different input states are scaled to the maximum of the corresponding row. The optical phase vortices have topological charge obeying the relation l = m s in − m s out .
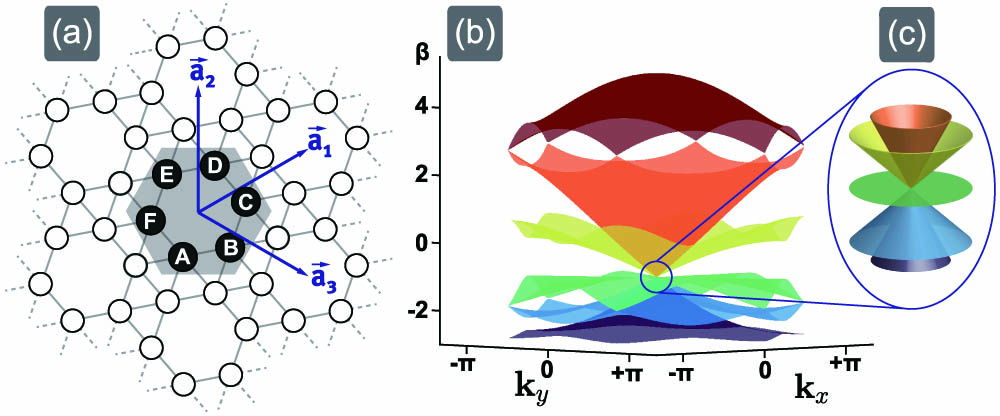
Set citation alerts for the article
Please enter your email address