Hanliu Zhao, Xinghao Sun, Zhengrui Zhu, Wen Zhong, Dongdong Song, Weibing Lu, Li Tao. Physical vapor deposited 2D bismuth for CMOS technology[J]. Journal of Semiconductors, 2020, 41(8): 081001

Search by keywords or author
- Journal of Semiconductors
- Vol. 41, Issue 8, 081001 (2020)
![(Color online) (a) Atomic structure of α phase P (left) and β phase As, Sb, Bi(right). Reproduced with permission from Ref. [4]. Copyright 2018, The Royal Society of Chemistry. (b) Top and side views of Bi(111), which exhibit a buckled honeycomb structure (left), and Bi(110), which exhibit a puckered black-phosphorus style allotrope. Reproduced with permission from Ref. [42]. Copyright 2020, The Royal Society of Chemistry. (c) Bulk Brillouin zone of Bi. Reproduced with permission from Ref. [20]. Copyright 2006, Elsevier Ltd. (d) Schematic drawing of the Bi band structure near the Fermi level at metallic (left) and semiconducting (right) phase. Reproduced with permission from Ref. [19]. Copyright 2006, American Physical Society.](/richHtml/jos/2020/41/8/081001/img_1.jpg)
Fig. 1. (Color online) (a) Atomic structure of α phase P (left) and β phase As, Sb, Bi(right). Reproduced with permission from Ref. [4 ]. Copyright 2018, The Royal Society of Chemistry. (b) Top and side views of Bi(111), which exhibit a buckled honeycomb structure (left), and Bi(110), which exhibit a puckered black-phosphorus style allotrope. Reproduced with permission from Ref. [42 ]. Copyright 2020, The Royal Society of Chemistry. (c) Bulk Brillouin zone of Bi. Reproduced with permission from Ref. [20 ]. Copyright 2006, Elsevier Ltd. (d) Schematic drawing of the Bi band structure near the Fermi level at metallic (left) and semiconducting (right) phase. Reproduced with permission from Ref. [19 ]. Copyright 2006, American Physical Society.
![(Color online) (a) Band structure of bismuthene is calculated without (up) and with (down) the SOC. Reproduced with permission from Ref. [39]. Copyright 2017, American Chemical Society. (b) Surface states of Bi (111) calculated without (black) and with (red) spin-orbit splitting included. The shaded areas show the projection of the bulk bands obtained without (violet) and with (yellow) SOC and their superposition (brown). (c) Calculated and measured electronic structure on Bi (111) surface. Reproduced with permission from Ref. [16]. Copyright 2004, American Physical Society.](/richHtml/jos/2020/41/8/081001/img_2.jpg)
Fig. 2. (Color online) (a) Band structure of bismuthene is calculated without (up) and with (down) the SOC. Reproduced with permission from Ref. [39 ]. Copyright 2017, American Chemical Society. (b) Surface states of Bi (111) calculated without (black) and with (red) spin-orbit splitting included. The shaded areas show the projection of the bulk bands obtained without (violet) and with (yellow) SOC and their superposition (brown). (c) Calculated and measured electronic structure on Bi (111) surface. Reproduced with permission from Ref. [16 ]. Copyright 2004, American Physical Society.
![(Color online) (a) Sketch and (b) STM image of flat honeycomb bismuthene epitaxy on SiC (0001). Reproduced with permission from Ref. [17]. Copyright 2020, American Association for the Advancement of Science. (c) STM image of ~ 1.2 monolayer Bi on Au (111) and the corresponding line profile (d) along the red line. Reproduced with permission from Ref. [56]. Copyright 2018, Elsevier B.V. (e) Topography of Bi nanoflakes, nanorods and nanoribbons grown on SiC buffer layer (BL) and epitaxy graphene (EG). (f) Atomic structure of Bi nanoribbon on EG. Reproduced with permission from Ref. [47]. Copyright 2018, American Chemical Society. (g) Phase diagram of the strain relief mechanism by interfacial misfit dislocations for epitaxy hetero-films. Reproduced with permission from Ref. [55]. Copyright 2020, AIP Publishing LLC.](/Images/icon/loading.gif)
Fig. 3. (Color online) (a) Sketch and (b) STM image of flat honeycomb bismuthene epitaxy on SiC (0001). Reproduced with permission from Ref. [17 ]. Copyright 2020, American Association for the Advancement of Science. (c) STM image of ~ 1.2 monolayer Bi on Au (111) and the corresponding line profile (d) along the red line. Reproduced with permission from Ref. [56 ]. Copyright 2018, Elsevier B.V. (e) Topography of Bi nanoflakes, nanorods and nanoribbons grown on SiC buffer layer (BL) and epitaxy graphene (EG). (f) Atomic structure of Bi nanoribbon on EG. Reproduced with permission from Ref. [47 ]. Copyright 2018, American Chemical Society. (g) Phase diagram of the strain relief mechanism by interfacial misfit dislocations for epitaxy hetero-films. Reproduced with permission from Ref. [55 ]. Copyright 2020, AIP Publishing LLC.
![(Color online) Morphology and X-ray-diffraction patterns (XRD) patterns of 2D bismuth deposited by PLD. (a) SEM micrographs of Bi films deposited by PLD at 185 °C on glass (left) and Si (100) (right). (b) Thickness-dependent XRD patterns of Bi films deposited at 20 °C on Si (100). Reproduced with permission from Ref. [63]. Copyright 1999, Elsevier Science B.V. (c) XRD patterns of the Bi thin films deposited under low ionic energy (~110 eV) and high ionic energy (~270 eV). Reproduced with permission from Ref. [62]. Copyright 2017, Elsevier B.V. (d) High-resolution TEM image of Bi (110) film and (e) Bi (111) film (scale bar = 2 nm), the inset is an enlarged image (scale bar = 1 nm). Reproduced with permission from Ref. [25]. Copyright 1999–2020, John Wiley & Sons, Inc.](/Images/icon/loading.gif)
Fig. 4. (Color online) Morphology and X-ray-diffraction patterns (XRD) patterns of 2D bismuth deposited by PLD. (a) SEM micrographs of Bi films deposited by PLD at 185 °C on glass (left) and Si (100) (right). (b) Thickness-dependent XRD patterns of Bi films deposited at 20 °C on Si (100). Reproduced with permission from Ref. [63 ]. Copyright 1999, Elsevier Science B.V. (c) XRD patterns of the Bi thin films deposited under low ionic energy (~110 eV) and high ionic energy (~270 eV). Reproduced with permission from Ref. [62 ]. Copyright 2017, Elsevier B.V. (d) High-resolution TEM image of Bi (110) film and (e) Bi (111) film (scale bar = 2 nm), the inset is an enlarged image (scale bar = 1 nm). Reproduced with permission from Ref. [25 ]. Copyright 1999–2020, John Wiley & Sons, Inc.
![(Color online) (a) X-ray reflectivity (XRR) curves for a 14 nm Bi (110) film grown at 40 K, measured at 300, 400 and 450 K. At 400 K, the onset of orientation transition towards a Bi (111) film is seen where at 450 K the entire film is transformed. (b) XRR curves for increasing Bi film thickness, grown at RT. Reproduced with permission from Ref. [65]. Copyright 2017, IOP Publishing Ltd. (c) XRD patterns of the bismuth thin films. From sample 1 to sample 3, the filament-substrate distance is decreased and film thickness increased. Reproduced with permission from Ref. [62]. Copyright 2017, Elsevier B.V.](/Images/icon/loading.gif)
Fig. 5. (Color online) (a) X-ray reflectivity (XRR) curves for a 14 nm Bi (110) film grown at 40 K, measured at 300, 400 and 450 K. At 400 K, the onset of orientation transition towards a Bi (111) film is seen where at 450 K the entire film is transformed. (b) XRR curves for increasing Bi film thickness, grown at RT. Reproduced with permission from Ref. [65 ]. Copyright 2017, IOP Publishing Ltd. (c) XRD patterns of the bismuth thin films. From sample 1 to sample 3, the filament-substrate distance is decreased and film thickness increased. Reproduced with permission from Ref. [62 ]. Copyright 2017, Elsevier B.V.
![(Color online) (a, b) Schematic diagram of the tube employed for the synthesis and atomic force microscopy image of 2D bismuth. Reproduced with permission from Ref. [24]. Copyright 2018, American Chemical Society. (c) Morphology evolution with substrate temperature. (d) The roughness of bismuth thin films prepared at different deposition temperatures. Reproduced with permission from Ref. [70]. Copyright 2019, Elsevier Ltd. (e) Electron concentration and mobility of flash evaporated bismuth thin films. (f) Oscillatory behavior of electrical resistivity. Reproduced with permission from Ref. [71]. Copyright 2007, Elsevier B.V.](/Images/icon/loading.gif)
Fig. 6. (Color online) (a, b) Schematic diagram of the tube employed for the synthesis and atomic force microscopy image of 2D bismuth. Reproduced with permission from Ref. [24 ]. Copyright 2018, American Chemical Society. (c) Morphology evolution with substrate temperature. (d) The roughness of bismuth thin films prepared at different deposition temperatures. Reproduced with permission from Ref. [70 ]. Copyright 2019, Elsevier Ltd. (e) Electron concentration and mobility of flash evaporated bismuth thin films. (f) Oscillatory behavior of electrical resistivity. Reproduced with permission from Ref. [71 ]. Copyright 2007, Elsevier B.V.
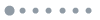
Fig. 7. (Color online) Schematic of 2D bismuth preferred orientation corresponding to different physical vapor deposition.
![(Color online) Transport properties characterization of PLD grown Bi (111) films. Reproduced with permission from Ref. [25]. Copyright 1999–2020, John Wiley & Sons, Inc. (a) The schematic of FETs based on Bi film. (b) Photograph of Bi FETs with patterned Au electrode (scale bar = 1 cm). The inset shows the optical picture of the electrode pattern of the device (scale bar = 100 μm). (c) Drain current-voltage output characteristics with gate bias at 0 V and (d) Drain current-gate voltage curve of the FET based on 10-nm-thick Bi film.](/Images/icon/loading.gif)
Fig. 8. (Color online) Transport properties characterization of PLD grown Bi (111) films. Reproduced with permission from Ref. [25 ]. Copyright 1999–2020, John Wiley & Sons, Inc. (a) The schematic of FETs based on Bi film. (b) Photograph of Bi FETs with patterned Au electrode (scale bar = 1 cm). The inset shows the optical picture of the electrode pattern of the device (scale bar = 100 μ m). (c) Drain current-voltage output characteristics with gate bias at 0 V and (d) Drain current-gate voltage curve of the FET based on 10-nm-thick Bi film.
![(Color online) (a) Three-dimensional schematic view of the Bi photodetector. (b) Time-dependent switching behavior of the photocurrent. Device area: 0.32 × 0.32 mm2. Power density: 300 mW/cm2. (c) Normalized responsivity as a function of illumination wavelength. Device size: 2 × 1.2 mm2. (d) Operating mechanism of the Bi photodetector. Reproduced with permission from Ref. [88]. Copyright 2015, Springer Nature. (e) Schematic view of the flexible Bi photodetector on the PI substrate. (f) Time-dependent photocurrent in the flexible photodetector excited by 808 nm laser with bias voltages of 2, 5, and 10 V. Reproduced with permission from Ref. [89]. Copyright 2020, American Chemical Society.](/Images/icon/loading.gif)
Fig. 9. (Color online) (a) Three-dimensional schematic view of the Bi photodetector. (b) Time-dependent switching behavior of the photocurrent. Device area: 0.32 × 0.32 mm2. Power density: 300 mW/cm2. (c) Normalized responsivity as a function of illumination wavelength. Device size: 2 × 1.2 mm2. (d) Operating mechanism of the Bi photodetector. Reproduced with permission from Ref. [88 ]. Copyright 2015, Springer Nature. (e) Schematic view of the flexible Bi photodetector on the PI substrate. (f) Time-dependent photocurrent in the flexible photodetector excited by 808 nm laser with bias voltages of 2, 5, and 10 V. Reproduced with permission from Ref. [89 ]. Copyright 2020, American Chemical Society.
![(Color online) (a−c) Formation process of all-optical switching based on XPM using 532 nm laser with intensity at 5.48 W/cm2. (d) Schematic experimental setup of XPM. Reproduced with permission from Ref. [90]. Copyright 2017, American Chemical Society. (e) Nonlinear transmittance and fitting curve. Reproduced with permission from Ref. [91]. Copyright 2018, The Royal Society of Chemistry. (f) Predicted zT value of bismuth thin films with different quantum wells. Reproduced with permission from Ref. [92]. Copyright 2020, AIP Publishing LLC. (g) Predicted zT value of monolayer bismuthene. Reproduced with permission from Ref. [93]. Copyright 2020, American Chemical Society.](/Images/icon/loading.gif)
Fig. 10. (Color online) (a−c) Formation process of all-optical switching based on XPM using 532 nm laser with intensity at 5.48 W/cm2. (d) Schematic experimental setup of XPM. Reproduced with permission from Ref. [90 ]. Copyright 2017, American Chemical Society. (e) Nonlinear transmittance and fitting curve. Reproduced with permission from Ref. [91 ]. Copyright 2018, The Royal Society of Chemistry. (f) Predicted zT value of bismuth thin films with different quantum wells. Reproduced with permission from Ref. [92 ]. Copyright 2020, AIP Publishing LLC. (g) Predicted zT value of monolayer bismuthene. Reproduced with permission from Ref. [93 ]. Copyright 2020, American Chemical Society.
![(Color online) (a) The second derivative of the Landau-level (SDLL) pattern of the 4.0-nm-thick film as a function of B. Acquisition conditions: –150 mV and 4 nA, modulation of 1.0 mV by root mean square. Reproduced with permission from Ref. [107]. Copyright 2016, Springer Nature. (b) Close-up of ARPES showing a valence-band maximum at the K point with large SOC-induced splitting in a wide momentum range. The sketch in the top-right corner depicts the orientation of the cut (black lines) in the momentum space of the SBZ (blue hexagon). (c) Differential conductivity dI/dV (reflecting the LDOS) at different distances to the edge. Reproduced with permission from Ref. [17]. Copyright 2020, American Association for the Advancement of Science. (d) Illustration of bismuthene/BP-Bi vertical homostructure on HOPG substrate. (e) STM image of a typical bismuthene island on BP-Bi layer. The red grid is superimposed in the right half of the bismuthene island to highlight the moiré superlattice. Scale bars, 40 Å. (f) dI/dV curves taken at three inequivalent sites at the edge and the center part of bismuthene island, respectively (set point: Vs = 0.3 V, I = 500 pA). Reproduced with permission from Ref. [108]. Copyright 2020, American Association for the Advancement of Science.](/Images/icon/loading.gif)
Fig. 11. (Color online) (a) The second derivative of the Landau-level (SDLL) pattern of the 4.0-nm-thick film as a function of B. Acquisition conditions: –150 mV and 4 nA, modulation of 1.0 mV by root mean square. Reproduced with permission from Ref. [107 ]. Copyright 2016, Springer Nature. (b) Close-up of ARPES showing a valence-band maximum at the K point with large SOC-induced splitting in a wide momentum range. The sketch in the top-right corner depicts the orientation of the cut (black lines) in the momentum space of the SBZ (blue hexagon). (c) Differential conductivity dI /dV (reflecting the LDOS) at different distances to the edge. Reproduced with permission from Ref. [17 ]. Copyright 2020, American Association for the Advancement of Science. (d) Illustration of bismuthene/BP-Bi vertical homostructure on HOPG substrate. (e) STM image of a typical bismuthene island on BP-Bi layer. The red grid is superimposed in the right half of the bismuthene island to highlight the moiré superlattice. Scale bars, 40 Å. (f) dI /dV curves taken at three inequivalent sites at the edge and the center part of bismuthene island, respectively (set point: V s = 0.3 V, I = 500 pA). Reproduced with permission from Ref. [108 ]. Copyright 2020, American Association for the Advancement of Science.
![(Color online) (a, b) Calculated temperature dependence of the magnetoresistance ratio for 29 nm and 193 nm bismuth thin films. Reproduced with permission from Ref. [110]. Copyright 2020, AIP Publishing LLC. (c) The temperature dependence of R and MR of bismuth thin film with thickness of 200 nm in 0 and 9 T, and (d) the magnetic field dependence of MR of bismuth thin film with different sample tilt angle θ at 300 K. Reproduced with permission from Ref. [111]. Copyright 2019, Elsevier Ltd.](/Images/icon/loading.gif)
Fig. 12. (Color online) (a, b) Calculated temperature dependence of the magnetoresistance ratio for 29 nm and 193 nm bismuth thin films. Reproduced with permission from Ref. [110 ]. Copyright 2020, AIP Publishing LLC. (c) The temperature dependence of R and MR of bismuth thin film with thickness of 200 nm in 0 and 9 T, and (d) the magnetic field dependence of MR of bismuth thin film with different sample tilt angle θ at 300 K. Reproduced with permission from Ref. [111 ]. Copyright 2019, Elsevier Ltd.
|
Table 1. Physical vapor deposition methods and corresponding parameters.

Set citation alerts for the article
Please enter your email address