
- Photonics Research
- Vol. 9, Issue 12, 2341 (2021)
Abstract
1. INTRODUCTION
Through technological advances, light-emitting diode (LED)-based systems have been commercialized and are now widely used in applications such as large displays, traffic lights, indoor and street lighting, automobiles, cell phones, and liquid-crystal displays [1–3]. Recently, white-light-emitting materials have attracted considerable attention owing to their potential use in LEDs, lighting, communications, and, especially, flexible display devices. White-light systems based on micro-LEDs and color converters such as quantum dots (QDs) are becoming more prominent in visible-light communication (VLC) applications. Furthermore, organic optoelectronic components are now being used in VLC systems because they are cheaper and more environmentally friendly, portable, and flexible than traditional silicon-based devices; moreover, they can be mass-produced [4–9]. Application of organic optoelectronic components to VLC systems will allow such systems to be incorporated into wearable fabrics and industrial products.
Regular white-light-emitting diodes (WLEDs) for lighting can be used for high-speed modulation; hence, they can be simultaneously applied to wireless data transmission at no major cost. However, WLED modulation bandwidths are significantly limited by the long response times of the color-conversion materials. Additionally, factors such as the dimming and flickering of color-conversion materials should be considered when designing modulation techniques. For example, in on–off keying (OOK) modulation techniques, LED devices are turned on or off depending on the data bit, where the on and off states present “1” and “0” bits, respectively. The shorter the fluorescence lifetime, the faster the luminous intensity drop to a characteristic point in the “0” state. For clear and rapid data representation, the switching time between “0” and “1” should be as short as possible, and the “0” and “1” luminous intensities should differ considerably; if such characteristics are realized, more data can be accurately reproduced in a unit time.
Full-color flexible displays can be fabricated using a flexible blue LED chip and color converters or yellow-light-emitting phosphors. However, the conventional white-light generation approach is unsuitable for flexible displays. Thus, inorganic white-light-emitting phosphors must be used, which are hard and brittle [10]. Quantum dots (QDs) have very promising application as color converters, especially because of their narrow full-width at half-maximum (FWHM) of approximately 30 nm; this affords high color purity and a tunable bandgap that is governed by the quantum size effect; further, the emission tunability is efficient and influenced by the QD composition [11–13]. Moreover, the non-radiative energy transfer between QDs and micro-LED enhances the color conversion efficiency of the full-color micro-LED devices [14]. Importantly, QD solutions can be cast on flexible substrates for use in flexible displays. For WLEDs, the fluorescence lifetimes of the color-conversion materials have a more significant influence on the resultant VLC. Unfortunately, the modulation bandwidth of common CdSe/ZnS QDs is limited to
Sign up for Photonics Research TOC. Get the latest issue of Photonics Research delivered right to you!Sign up now
Halide perovskite QDs (HPQDs) have evolved as an alternative to CdSe/ZnS QDs because of their outstanding characteristics, which derive from their narrower FWHM (below 30 nm). This property yields greater color purity, higher photoluminescence quantum yield (approaching 100%), more convenient synthesis, and lower manufacturing costs than conventional QDs [16,17]. In particular, PQDs exhibiting short carrier recombination lifetimes and narrow spectral linewidths have attracted interest because they can be used to develop white-light VLC systems with considerably higher modulation bandwidth than CdSe/ZnS QDs [18]. Therefore, HPQDs have been used in different electronic and optoelectronic applications, such as in photodetection, photovoltaics, and photoemission; however, they are usually employed as active materials or color converters in LEDs [19–22].
The highest reported modulation bandwidth for a white-light system combining a micro-LED with PQDs is low, at 85 MHz [23]. Therefore, increasing the bandwidth of the VLC white-light source is essential, and considerable efforts have been made to increase the bandwidths of both color converters and blue LEDs [24,25]. Recently, Xia
In this study, we propose a high-performance flexible white-light system that has immense potential for VLC applications. This proposed system is constructed with nanostructured green-emitting
2. EXPERIMENT
A. Preparation of the Cellulose Nanocrystal Suspension
To prepare the cellulose nanocrystal suspension, 168 mg freeze-dried cellulose nanocrystals (CNCs) were mixed with 16 mL anhydrous dimethylformamide solution. The mixed solution was then sonicated for approximately 2 h.
B. Preparation of the
To prepare the Cs–oleate solution, 1.05 g
C. Preparation of the CdSe QD Paper
To prepare the CdSe QDs, 1 mmol CdO, 15 mL ODE, and 1.44 mmol OA were mixed and then heated to 300°C under a
D. Fabrication of the Semipolar Micro-LED Device
The structure and fabrication process of the micro-LED device were similar to those employed in our previous studies [28–30]. Metal organic chemical vapor deposition (MOCVD) was used to grow semipolar (20–21)-oriented GaN layers on patterned sapphire substrates. To directly develop GaN on a sapphire wafer, we employed an advanced orientation-controlled epitaxy method; this is a simple epitaxy technique involving MOCVD for developing semipolar GaN.
For c-plane devices, a large polarization field exists across the quantum wells (QWs). This causes a severe quantum-confined stark effect (QCSE), resulting in less overlap of the electron–hole wave function and an increased recombination lifetime. These issues can be overcome by growing semipolar planes with a high inclination angle, such as (20–21) [31]. Semipolar blue micro-LEDs have gained substantial attention owing to their specific mechanical properties and device performance, such as critical thickness, high efficiency, low efficiency droop, and low thermal droop [32–34]. In addition, both the efficiency droop and thermal droop can be minimized by the growth of thick single-QW (SQW) structures of semipolar planes.
In this study, semipolar (20–21) GaN was developed after the substrates were prepared. Ge doping was then employed in the initial epitaxy phase to produce stacking-fault-free semipolar GaN. A bulk GaN layer was formed by depositing undoped GaN after the Ge-doped GaN strips had coalesced. The micro-LED epitaxial structure consisted of an n-GaN layer, an InGaN/GaN SQW as the active region, and a p-type GaN layer. The micro-LED was fabricated through deposition of an indium tin oxide (ITO) layer, followed by mesa etching of the ITO film using hydrochloric acid and an inductively coupled plasma reactive ion-etching process (to form a p-type ohmic contact). This process was accompanied by thermal annealing to provide a better current-spreading layer. Finally, the electrodes were deposited with a Ti/Al/Ti/Au structure, after which an
Figure 1.(a) Schematic of semipolar micro-LED structure; (b) optical microscopy image of micro-LED.
E. Fabrication of the Flexible White-Light System
A flexible white-light system was developed using a flexible blue micro-LED and
Figure 2.Fabrication of white-light system.
3. RESULTS AND DISCUSSION
Figure 3 shows the structural and optical characteristics of the fabricated PQD paper. In contrast to the standard phase of
Figure 3.(a) XRD patterns, (b) TEM images; inset:
The optical characteristics of the fabricated micro-LED, PQD paper, and white-light system were analyzed. The light–current–voltage (L–I–V) curve for the semipolar micro-LED at a 25°C operating temperature is shown in Fig. 4(a). The semipolar micro-LED had a forward voltage of approximately 3.45 V, suggesting electrical characteristics comparable to those of c-plane devices. In detail, this micro-LED had 4.5 mW light output power at a 72 mA injection current. The light output power produced by the semipolar micro-LED was sufficient to pump the PQD paper and CdSe QD paper to realize the white-light system. Figure 4(a) inset is an optical image of the illumination from the semipolar micro-LED array. The electroluminescence (EL) spectra of the semipolar micro-LED attained under different driving currents are shown in Fig. 4(b). A 441 nm peak emission wavelength and a narrow FWHM of 28.19 nm were observed under a 72 mA injection current. This narrow FWHM suggests that the epitaxy produced a high-quality structure and was therefore responsible for the delivery of pure emitted light, matching colors, and a wide color gamut. This outcome indicates that the epitaxial features of the semipolar plane were similar to those of c-plane devices. However, the FWHM increased with the current, possibly because of the band-filling effect in the SQW. A slight blueshift in the EL spectrum was observed as the injection current increased from 7.2 mA; the wavelength stabilized after 72 mA. This blueshift can be attributed to the reduced polarization-related electric fields in the SQWs grown on the semipolar orientation, which reduced the QCSE.
Sign up for Photonics Research TOC. Get the latest issue of Photonics Research delivered right to you!Sign up now
Figure 4.(a)
The peak wavelength shifts of the c-plane and semipolar micro-LEDs in response to injection current density variation from 1 to
Time-resolved photoluminescence (TRPL) was employed to investigate the carrier lifetimes of both the semipolar micro-LED and PQD paper as shown in Fig. 4(d). The photoluminescence (PL) decay curves were fitted using a double-exponential decay function, and the average lifetime (
To realize a flexible white-light system, green-emitting PQD paper and red-emitting CdSe QD paper were used to form a color converter and placed on top of the semipolar blue micro-LED with flexible substrate. Figure 5(a) shows the EL spectrum of the white light generated from the flexible white-light system as the micro-LED drive current increased from 7.2 to 72 mA. This spectrum also shows the green and red light emissions from the PQD and CdSe QD papers, respectively, which had peak wavelengths of 528 and 625 nm, respectively; these results confirm the white-light generation. Figure 5(a) inset shows a flexible white-light system with high white-light illumination suitable for application in VLC wearable devices.
Figure 5.(a) White-light spectrum generated using semipolar micro-LED and PQD and CdSe QD papers. Inset: photograph of flexible white-light system. (b) Color gamut of white-light system according to CIE 1931 color space under various current densities.
In addition to its potential for VLC applications, the PQD-based flexible white-light system reported herein displays a wide range of color characteristics and excellent color stability, which is very promising for display applications. Figure 5(b) shows the color performance of the white-light system created using the semipolar blue micro-LED with PQD paper and CdSe QD paper under driving conditions from 10 to
The experimental setup used to measure the 3 dB modulation bandwidth is shown in Fig. 6. The measurement was performed using a vector network analyzer (Keysight E5071C) by supplying an alternating current to a direct-current bias tee, which was used to drive the micro-LED. The micro-LED then pumped the
Figure 6.Schematic of experimental setup for VLC bandwidth measurement.
Figure 7 shows the results of the frequency response measurement and the extracted 3 dB modulation bandwidth for the flexible semipolar micro-LED, PQD paper, CdSe QD paper, and white-light system with increasing injection current at a transmission distance of 25 cm. The 3 dB bandwidth increased with the injection current; this can be attributed to the carrier lifetime reduction at higher injection current density. For the semipolar micro-LED, the carrier lifetime decreased with increasing injection current density in the active region, owing to the built-in electric-field screening effect. The semipolar blue micro-LED had a maximum 3 dB bandwidth of 817 MHz corresponding to an injection current of 113 mA as shown in Fig. 7(a); this is promising for VLC applications.
Figure 7.(a) Frequency responses for semipolar blue micro-LED; inset: eye diagram. (b) Comparison of bandwidth of PQD paper in nanostructure with that of PQD film; inset: eye diagram for PQD paper.
The measured modulation bandwidth for the semipolar blue micro-LED was higher than that of the green-emitting semipolar micro-LED [34]. The short average carrier lifetime of the semipolar micro-LED was consistent with the measured frequency bandwidth. The eye diagram of the semipolar micro-LED is shown in Fig. 7(a) inset. A maximum data rate of 1.5 Gbps was achieved owing to the sufficiently high 3 dB bandwidth. Note that, for micro-LEDs, the carrier recombination lifetime is related to the recombination rate, as a higher recombination rate shortens the carrier lifetime. The relationship is expressed as
The differential carrier lifetime
The TRPL data indicate the carrier lifetime, but not the differential carrier lifetime; however, the latter is usually 2–3 times shorter than the former. Consequently, a shorter carrier lifetime induces a shorter differential carrier lifetime and, as a result, a greater modulation bandwidth. Therefore, the high bandwidths of the semipolar micro-LED and PQD paper can be successfully explained by referring to the above explanation.
In a comparison of the PQD paper prepared with cellulose with a typical PQD film prepared through film-casting, the bandwidth of the nanostructured PQD paper was much higher than that of the PQD film as shown in Fig. 7(b). The method for the preparation of
Table 1 summarizes the results of studies investigating the use of QDs for VLC; the QD bandwidths are not remarkably high. Figure 8 shows various results for the 3 dB bandwidth benchmark as a function of current, as many researchers have attempted to increase the QD bandwidth. For example, Leitão
Overview of Reported QDs for VLC
Materials Type | Decay Lifetime (τ) | Bandwidth | Data Rate | References |
---|---|---|---|---|
5.92 ns | 229 MHz | 400 Mbps | This work | |
5.93 ns | 180 MHz | 185 Mbps | [ | |
43.74 ns | 70 MHz | 150 Mbps | [ | |
– | 24.6 MHz | 364 Mbps | [ | |
77 ns | 5.4 MHz | – | [ | |
CdSe/ZnS QDs | 17.24 ns | 23.1 MHz | – | [ |
7 ns | 41 MHz | 380 Mbps | [ | |
CdSe/ZnS QDs | 26.31 ns | 2.70 MHz | – | [ |
Figure 8.Benchmark of 3 dB bandwidth for QDs with applied current.
The use of a white-light system for VLC applications has been reported in various studies. In 2018, Mei
4. CONCLUSIONS
In this study, we demonstrated the potential of nanostructured green
Acknowledgment
Acknowledgment. The authors thank Pengfei Tian of Fudan University for the helpful discussion.
References
[1] J. Cho, J. H. Park, J. K. Kim, E. F. Schubert. White light-emitting diodes: history, progress, and future. Laser Photon. Rev., 11, 1600147(2017).
[2] M. D. Al-Amri, D. Zhu, M. El-Gomati, C. J. Humphreys, M. S. Zubairy. Solid-state lighting based on light emitting diode technology. Optics in Our Time, 87-118(2016).
[3] A. Žukauskas, M. S. Shur, R. Gaska. Light-emitting diodes: progress in solid-state lighting. MRS Bull., 26, 764-769(2001).
[4] B. Arredondo, B. Romero, J. M. Sánchez Pena, A. Fernández-Pacheco, E. Alonso, R. Vergaz, C. de Dioz. Visible light communication system using an organic bulk heterojunction photodetector. Sensors, 13, 12266-12276(2013).
[5] P. A. Haigh, F. Bausi, H. Le Minh, I. Papakonstantinou, W. O. Popoola, A. Burton, F. Cacialli. Wavelength-multiplexed polymer LEDs: towards 55 Mb/s organic visible light communications. IEEE J. Sel. Areas Commun., 33, 1819-1828(2015).
[6] P. A. Haigh, Z. Ghassemlooy, S. Rajbhandari, I. Papakonstantinou. Visible light communications using organic light emitting diodes. IEEE Commun. Mag., 51, 148-154(2013).
[7] M. T. Sajjad, P. P. Manousiadis, H. Chun, D. A. Vithanage, S. Rajbhandari, A. L. Kanibolotsky, G. Faulkner, D. O’Brien, P. J. Skabara, I. D. W. Samuel, G. A. Turnbull. Novel fast color-converter for visible light communication using a blend of conjugated polymers. ACS Photon., 2, 194-199(2015).
[8] L. Wang, Z. Wei, C.-J. Chen, L. Wang, H. Y. Fu, L. Zhang, K.-C. Chen, M.-C. Wu, Y. Dong, Z. Hao, Y. Luo. 1.3 GHz E-O bandwidth GaN-based micro-LED for multi-gigabit visible light communication. Photon. Res., 9, 792-802(2021).
[9] S. Zhang, D. Tsonev, S. Videv, S. Ghosh, G. A. Turnbull, I. D. W. Samuel, H. Haas. Organic solar cells as high-speed data detectors for visible light communication. Optica, 2, 607-610(2015).
[10] Y. Xu, J. Chen, H. Zhang, H. Wei, L. Zhou, Z. Wang, Y. Pan, X. Su, A. Zhang, J. Fu. White-light-emitting flexible display devices based on double network hydrogels crosslinked by YAG:Ce phosphors. J. Mater. Chem. C, 8, 247-252(2020).
[11] X. Dai, Z. Zhang, Y. Jin, Y. Niu, H. Cao, X. Liang, L. Chen, J. Wang, X. Peng. Solution-processed, high-performance light-emitting diodes based on quantum dots. Nature, 515, 96-99(2014).
[12] Y.-M. Huang, K. James Singh, A.-C. Liu, C.-C. Lin, Z. Chen, K. Wang, Y. Lin, Z. Liu, T. Wu, H.-C. Kuo. Advances in quantum-dot-based displays. Nanomaterials, 10, 1327(2020).
[13] V. Wood, V. Bulović. Colloidal quantum dot light-emitting devices. Nano Rev., 1, 5202(2010).
[14] X. L. Fan, T. Wu, B. Liu, R. Zhang, H. C. Kuo, Z. Chen. Recent developments of quantum dot based micro-LED based on non-radiative energy transfer mechanism. Opto-Electron Adv., 4, 21002201(2021).
[15] X. Xiao, H. Tang, T. Zhang, W. Chen, W. Chen, D. Wu, R. Wang, K. Wang. Improving the modulation bandwidth of LED by CdSe/ZnS quantum dots for visible light communication. Opt. Express, 24, 21577-21586(2016).
[16] C.-Y. Kang, C.-H. Lin, C.-H. Lin, T.-Y. Li, S.-W. Huang Chen, C.-L. Tsai, C.-W. Sher, T.-Z. Wu, P.-T. Lee, X. Xu, M. Zhang, C.-H. Ho, J.-H. He, H.-C. Kuo. Highly efficient and stable white light-emitting diodes using perovskite quantum dot paper. Adv. Sci., 6, 1902230(2019).
[17] Q. V. Le, K. Hong, H. W. Jang, S. Y. Kim. Halide perovskite quantum dots for light-emitting diodes: properties, synthesis, applications, and outlooks. Adv. Electron. Mater., 4, 1800335(2018).
[18] I. Dursun, C. Shen, M. R. Parida, J. Pan, S. P. Sarmah, D. Priante, N. Alyami, J. Liu, M. I. Saidaminov, M. S. Alias, A. L. Abdelhady, T. K. Ng, O. F. Mohammed, B. S. Ooi, O. M. Bakr. Perovskite nanocrystals as a color converter for visible light communication. ACS Photon., 3, 1150-1156(2016).
[19] C. Jiang, J. Yao, P. Huang, R. Tang, X. Wang, X. Lei, H. Zeng, S. Chang, H. Zhong, H. Yao, C. Zhu, T. Chen. Perovskite quantum dots exhibiting strong hole extraction capability for efficient inorganic thin film solar cells. Cell Rep. Phys. Sci., 1, 100001(2020).
[20] P. Ramasamy, D.-H. Lim, B. Kim, S.-H. Lee, M.-S. Lee, J.-S. Lee. All-inorganic cesium lead halide perovskite nanocrystals for photodetector applications. Chem. Commun., 52, 2067-2070(2016).
[21] S. A. Veldhuis, P. P. Boix, N. Yantara, M. Li, T. C. Sum, N. Mathews, S. G. Mhaisalkar. Perovskite materials for light-emitting diodes and lasers. Adv. Mater., 28, 6804-6834(2016).
[22] X. Zhang, H. Liu, W. Wang, J. Zhang, B. Xu, K. L. Karen, Y. Zheng, S. Liu, S. Chen, K. Wang, X. W. Sun. Hybrid perovskite light-emitting diodes based on perovskite nanocrystals with organic–inorganic mixed cations. Adv. Mater., 29, 1606405(2017).
[23] S. Mei, X. Liu, W. Zhang, R. Liu, L. Zheng, R. Guo, P. Tian. High-bandwidth white-light system combining a micro-LED with perovskite quantum dots for visible light communication. ACS Appl. Mater. Interfaces, 10, 5641-5648(2018).
[24] N. Laurand, B. Guilhabert, J. McKendry, A. E. Kelly, B. Rae, D. Massoubre, Z. Gong, E. Gu, R. Henderson, M. D. Dawson. Colloidal quantum dot nanocomposites for visible wavelength conversion of modulated optical signals. Opt. Mater. Express, 2, 250-260(2012).
[25] H. Li, X. Chen, J. Guo, H. Chen. A 550 Mbit/s real-time visible light communication system based on phosphorescent white light LED for practical high-speed low-complexity application. Opt. Express, 22, 27203-27213(2014).
[26] M. Xia, S. Zhu, J. Luo, Y. Xu, P. Tian, G. Niu, J. Tang. Ultrastable perovskite nanocrystals in all-inorganic transparent matrix for high-speed underwater wireless optical communication. Adv. Opt. Mater., 9, 2002239(2021).
[27] K. James Singh, Y.-M. Huang, T. Ahmed, A.-C. Liu, S.-W. Huang Chen, F.-J. Liou, T. Wu, C.-C. Lin, C.-W. Chow, G.-R. Lin, H.-C. Kuo. Micro-LED as a promising candidate for high-speed visible light communication. Appl. Sci., 10, 7384(2020).
[28] S.-W. H. Chen, Y.-M. Huang, Y.-H. Chang, Y. Lin, F.-J. Liou, Y.-C. Hsu, J. Song, J. Choi, C.-W. Chow, C.-C. Lin, R.-H. Horng, Z. Chen, J. Han, T. Wu, H.-C. Kuo. High-bandwidth green semipolar (20–21) InGaN/GaN micro light-emitting diodes for visible light communication. ACS Photon., 7, 2228-2235(2020).
[29] S.-W. H. Chen, Y.-M. Huang, K. James Singh, Y.-C. Hsu, F.-J. Liou, J. Song, J. Choi, P.-T. Lee, C.-C. Lin, Z. Chen, J. Han, T. Wu, H.-C. Kuo. Full-color micro-LED display with high color stability using semipolar (20–21) InGaN LEDs and quantum-dot photoresist. Photon. Res., 8, 630-636(2020).
[30] S.-W. Huang Chen, C.-C. Shen, T. Wu, Z.-Y. Liao, L.-F. Chen, J.-R. Zhou, C.-F. Lee, C.-H. Lin, C.-C. Lin, C.-W. Sher, P.-T. Lee, A.-J. Tzou, Z. Chen, H.-C. Kuo. Full-color monolithic hybrid quantum dot nanoring micro light-emitting diodes with improved efficiency using atomic layer deposition and nonradiative resonant energy transfer. Photon. Res., 7, 416-422(2019).
[31] Y. H. Chang, Y. M. Huang, W. H. Gunawan, G. H. Chang, F. J. Liou, C. W. Chow, H. C. Kuo, Y. Liu, C. H. Yeh. 4.343-Gbit/s green semipolar (20-21) μ-LED for high speed visible light communication. IEEE Photon. J., 13, 7300204(2021).
[32] H. Fu, Z. Lu, X.-H. Zhao, Y.-H. Zhang, S. P. DenBaars, S. Nakamura, Y. Zhao. Study of low-efficiency droop in semipolar (20-2-1) InGaN light-emitting diodes by time-resolved photoluminescence. J. Disp. Technol., 12, 736-741(2016).
[33] M. Monavarian, A. Rashidi, A. Aragon, S. Oh, M. Nami, S. DenBaars, D. Feezell. Explanation of low efficiency droop in semipolar (20-2-1) InGaN/GaN LEDs through evaluation of carrier recombination coefficients. Opt. Express, 25, 19343-19353(2017).
[34] C. Shen, T. Ng, J. Leonard, A. Pourhashemi, S. Nakamura, S. DenBaars, J. Speck, A. Alyamani, M. El-Desouki, B. Ooi. High-brightness semipolar (202-1) blue InGaN/GaN superluminescent diodes for droop-free solid-state lighting and visible-light communications. Opt. Lett., 41, 2608-2611(2016).
[35] D.-H. Lee, J.-H. Lee, J.-S. Park, T.-Y. Seong, H. Amano. Improving the leakage characteristics and efficiency of GaN-based micro-light-emitting diode with optimized passivation. ECS J. Solid State Sci. Technol., 9, 055001(2020).
[36] M. S. Wong, D. Hwang, A. I. Alhassan, C. Lee, R. Ley, S. Nakamura, S. P. DenBaars. High efficiency of III-nitride micro-light-emitting diodes by sidewall passivation using atomic layer deposition. Opt. Express, 26, 21324-21331(2018).
[37] Y.-H. Chang, J.-C. Lin, Y.-C. Chen, T.-R. Kuo, D.-Y. Wang. Facile synthesis of two-dimensional Ruddlesden–Popper perovskite quantum dots with fine-tunable optical properties. Nanoscale Res. Lett., 13, 247(2018).
[38] L. Zhou, K. Yu, F. Yang, H. Cong, N. Wang, J. Zheng, Y. Zuo, C. Li, B. Cheng, Q. Wang. Insight into the effect of ligand-exchange on colloidal CsPbBr3 perovskite quantum dot/mesoporous-TiO2 composite-based photodetectors: much faster electron injection. J. Mater. Chem. C, 5, 6224-6233(2017).
[39] M. F. Leitão, M. S. Islim, L. Yin, S. Viola, S. Watson, A. Kelly, X. Li, D. Yu, H. Zeng, S. Videv, H. Haas, E. Gu, N. Laurand, M. D. Dawson. MicroLED-pumped perovskite quantum dot color converter for visible light communications. IEEE Photonics Conference (IPC), 69-70(2017).
[40] C. Ruan, Y. Zhang, M. Lu, C. Ji, C. Sun, X. Chen, H. Chen, V. L. Colvin, W. W. Yu. White light-emitting diodes based on AgInS2/ZnS quantum dots with improved bandwidth in visible light communication. Nanomaterials, 6, 13(2016).
[41] H. Cao, S. Lin, Z. Ma, X. Li, J. Li, L. Zhao. Color converted white light-emitting diodes with 637.6 MHz modulation bandwidth. IEEE Electron Device Lett., 40, 267-270(2019).
[42] M. Leitão, M. Islim, L. Yin, S. Viola, S. Watson, A. Kelly, Y. Dong, X. Li, H. Zeng, S. Videv, H. Haas, E. Gu, I. Watson, N. Laurand, M. Dawson. Pump-power-dependence of a CsPbBr3-in-Cs4PbBr6 quantum dot color converter. Opt. Mater. Express, 9, 3504-3518(2019).
[43] C. Yeh, Y. Liu, C. Chow. Real-time white-light phosphor-LED visible light communication (VLC) with compact size. Opt. Express, 21, 26192-26197(2013).
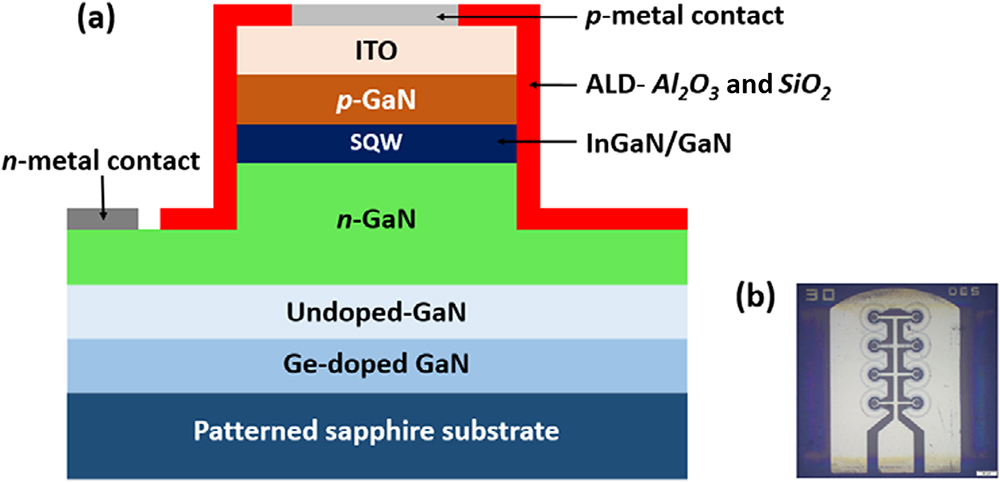
Set citation alerts for the article
Please enter your email address