
- Photonics Research
- Vol. 10, Issue 7, 1650 (2022)
Abstract
1. INTRODUCTION
Free-electron-based sciences, technologies, and installations have demonstrated enormous potential in fundamental physics, devices, and applications of photonics and optoelectronics [1–3]. The interactions between free electrons and materials or metamaterials can be used to generate radiation from the terahertz to the ultraviolet band [4,5]. The most widely known free-electron radiations include Cherenkov radiation (CR) [6–8], transition radiation [9,10], Smith–Purcell radiation [11], synchrotron radiation, and bremsstrahlung. Free-electron radiation depends not only on the parameters of free electrons but also on the complex local or nonlocal properties of the electromagnetic medium. Thus, we can control free-electron radiation by manipulating the environment (e.g., materials or structures) around electrons [12].
CR is one of the most widely studied categories of free-electron radiation. The earliest CR experiments were performed by Cherenkov and Vavilov in 1934 [13,14]. Then, their experimental results were theoretically explained by Tamm and Frank in 1937 [15]. CR is generated when the electron (or particle) velocity,
Over the past two decades, the utilization of different natural materials and metamaterials has reignited research on CR [12]. For example, we found that surface plasmon polaritons supported by a noble metal film (e.g., Ag) excited by free electrons can be transformed into intense and coherent CR in the visible and ultraviolet bands [20]. However, a relatively high electron velocity (i.e.,
Sign up for Photonics Research TOC. Get the latest issue of Photonics Research delivered right to you!Sign up now
Besides, interesting CR phenomena can also be elicited in photonic crystals or designed metamaterials [27,28]. We have already demonstrated that reversed CR can be obtained experimentally from a left-handed material in the microwave band with opposite directions between the wave vector and energy flux [29]. In addition to CR properties, we also devised a new mechanism of direction-controllable inverse transition radiation in a graphene multilayer structure working in the terahertz and mid-infrared bands [30]. Compared with the general case, transition radiation generated in this structure is dramatically enhanced. Recently, we also found that in-plane CR can be generated efficiently in the graphene hyperbolic grating, achieving a stronger CR than that generated in normal graphene-based HMMs (GHMs) [31].
Considering different topological materials and structures, topological photonics is being rapidly developed and can be applied to control the properties of light in different media [32,33]. For example, the optical topological transition (OTT) in some metamaterials enables innovative and effective control [32–34]. The OTT can also appear in HMMs and natural hyperbolic materials [34–36]. If the OTT occurs in an HMM, its topological iso-frequency surface can transform from ellipsoid to hyperboloid and vice versa. This phenomenon can be applied to manipulate light–matter interactions in HMMs, such as the spontaneous emission rate of nearby emitters [34]. With the development of theoretical and experimental techniques in recent years, the OTT can also be induced in natural materials by external ultrafast optical pump beams [36]. Thus, the topology phase of the iso-frequency surface of GHMs can be switched between ellipsoid and hyperboloid in an ultrafast manner.
In this study, we determined the importance of the OTT to generate and manipulate CR in GHMs, in which the OTT frequency,
2. METHOD
In this section, we present the methods to analyze the GHM properties and then calculate and explain several phenomena related to the OTT of CR in the GHM. First, the basic structure of the studied GHM for this work is described. Then, the macroscopic EMT and microscopic SMT are detailed. Finally, three widely used graphene conductivity models are presented, including the classical Drude model, photothermal (temperature-dependent) model, and nonlocal model (wave-vector-dependent), which is based on random-phase approximation with relaxation-time approximation. Note that, among these three models, only the last model considers momentum- or wave-vector-dependent effects, but just the function of frequency.
A. GHM
We use a graphene-dielectric multilayer structure as the platform of the HMM for CR because graphene provides numerous advantages as the metallic part of the HMM [37]. Remarkably, graphene possesses excellent tunable electrical/optical properties, broadband and ultrafast optical response (especially in the terahertz and mid-infrared bands), high electron mobility and low energy consumption [38], and wave-vector-dependent or nonlocal property [39]. As a result, GHMs can establish a promising platform for tunable CR-based sources, detectors, and devices.
The proposed GHM for CR is illustrated in Fig. 1. Free electrons with constant velocity
Figure 1.CR excited by low-energy electrons in the GHM. Features of the hyperbolic CR in the hyperbolic state (left half) and conventional CR in the elliptical state (right half).
B. Macroscopic EMT
The thicknesses of the graphene and dielectric buffer layers in our GHM are much lower than the considered wavelengths. Therefore, the GHM can be approximated as a homogeneous anisotropic medium described by the macroscopic EMT [40], which provides its effective in-plane (parallel) and out-of-plane (perpendicular) permittivity components.
The effective permittivity component of the GHM can be approximated using previous results [41,42]. By the EMT, we can analyze the OTT of CR generated in the GHM, including the effective in-plane and out-of-plane permittivity components,
C. Microscopic SMT
From the microscopic perspective, the dispersion relation and field distribution of plasmon modes inside the GHM can be calculated by rigorously applying the SMT. According to the SMT, multiple plasmon modes inside the GHM can be excited flexibly by altering the electron velocities. Interactions between these plasmon modes are complicated and make them deviate from their original positions. Effective hyperbolic CR generated in the GHM is the superposition of coupled plasmon modes, especially acoustic modes.
By adapting the existing SMT [43,44], we calculate the imaginary part of the reflection coefficient,
Figure 2.Basic principles of SMT. T and R represent the transmission and reflection coefficients, respectively. The purple and red boxes indicate the connection of scattering matrices in the interface and inside the region, respectively.
In the SMT, each interface and region is represented by a
D. Conductivity Model of Graphene
The most used conductivity model of graphene is the classical Drude model, which only considers intraband electron transition and often neglects temperature-dependent effects in highly doped graphene [37,39]. However, the chemical potential of graphene depends on the temperature of graphene electrons when it is high enough. With highly efficient and ultrafast thermodynamic properties, electrons can be heated instantaneously when an ultrafast laser illuminates graphene, while the temperature of crystal lattice remains almost unchanged. Therefore, the photothermal properties of graphene optical conductivity should be described by a modified model involving electron temperature [35,45]. In this work, we suggest that graphene photothermal conductivity can be used to analyze the ultrafast OTT of CR in the GHM. It can be caused under the illumination of an external optical pump, as explained in Section 3.
On the other hand, when the electron velocity is low enough, it causes a large wave vector. When this happens, graphene nonlocality should be considered. Based on the widely used nonlocal random-phase approximation graphene nonlocal conductivity model, which neglects the interaction between graphene electrons, we investigate the effects of graphene nonlocality on the OTT of CR in the GHM. In addition to dispersive modifications, it also brings a new lower velocity threshold to achieve the OTT for CR. This velocity threshold is roughly equal to the Fermi velocity of graphene, at which electrons enter the electron-hole continuum or Landau damping region [39]. Strictly, the nonlocal phenomena of quantum materials should be analyzed by further theoretical methods, such as quantum-corrected hydrodynamics and microscopic surface-response functions, which are beyond the scope of this work.
3. RESULTS AND DISCUSSION
In this section, we first use the classical Drude model for analysis. Then, the photothermal and nonlocal models are used to analyze the electron temperature- and wave-vector-dependent effects of CR in the GHM.
The EMT allows for describing the effective permittivity components of the GHM accurately. Specifically, the effective permittivity of graphene,
In addition, the effective in-plane and out-of-plane permittivities of the GHM can be well described as [41,42]
The effective reflective index
According to the EMT, the real part of the effective in-plane permittivity,
Figure 3.Effective in-plane permittivity of the GHM described by the EMT.
With the effective in-plane permittivity components [Figs. 3(a) and 3(b)], we can obtain
If the electron velocity is lower or higher than the phase velocity of light in the dielectric buffer layer,
Shortly, in the GHM,
We can also obtain the directions for effective CR, including wave vector
Figure 4.Effective CR properties. (a) Directions of wave vector
The normalized effective CR spectrum is shown in Fig. 4(b). There exist two regions for CR. For high-energy electrons (
The EMT allowed us to demonstrate that the GHM, which serves as a tunable HMM, can be used in principle as an ideal platform for CR generation using low-energy electrons. The main parameter that determines the generation of CR inside the GHM is the OTT frequency
To determine the specific behavior inside the GHM, we should calculate the supported plasmonic modes and dispersion properties of the GHM by an optical matrix method such as the SMT [43,44]. The SMT provides the imaginary part of the reflection coefficient,
Figure 5.(a) Dispersion of plasmon modes in the GHM characterized by the imaginary part of reflection coefficient,
If the plasmon modes have larger wave vectors, they are weaker and more difficult to be excited. For example, except for the strongest optical plasmon mode [the rightmost one in Fig. 5(a)], other plasmon modes are weaker. The most intense position of
Suitable GHM topological states and electron velocity should be simultaneously provided to ensure CR generation. If the system locates in the hyperbolic state, the plasmon modes can be excited by low-energy electrons, and effective hyperbolic CR can be generated. In contrast, if it exhibits elliptical properties, the plasmon modes cannot be excited, and CR can only be induced by high-energy electrons. From the plasmon dispersion, we can analyze the OTT of CR in the GHM from the hyperbolic state to the elliptical state. In Fig. 5(b), we select some frequencies below and above
The field distribution of graphene plasmon modes indicates that effective CR generation is essentially the superposition of coupled propagating plasmon modes excited in each graphene layer, which notably differs from the mechanism of conventional CR generated in normal media. By the SMT, the representative spatial field distribution of plasmon modes in the GHM is shown in Fig. 6 and corresponds to the points labeled by white letters in Fig. 5(a), in which we also indicate the directions of effective CR calculated by the EMT [Figs. 6(c)–6(f)]. The white dashed lines in Fig. 6 indicate the positions of graphene layers.
Figure 6.Electric field spatial distributions of excited plasmon modes parallel to the
For frequencies above
For frequencies below
Although the properties of hyperbolic CR can be altered by various parameters of the GHM, active optical manipulating methods of CR remain to be devised. Instead of electrical tuning of the Fermi level of graphene, we propose the concept of an ultrafast CR source by leveraging the photothermal effect of graphene [35,45]. The temperature-dependent effects of graphene electrons can be used to induce ultrafast OTT in the GHM given the extremely small electronic heat capacity of graphene. On the other hand, conventional electrical tuning is difficult to implement in a multilayer structure, rendering optical modulation of graphene properties more attractive.
Hot carriers in graphene are generated almost instantaneously in an ultrafast response caused by an external ultrafast optical pump [35,45]. Ultrafast photothermal properties can be obtained owing to advantages of graphene such as low carrier density and high carrier mobility, low electronic heat capacity, large variation of the optical response induced by electronic heating, and relatively weak electron-phonon interactions, all of which result in the ultrafast and substantial photothermal response of graphene. With an optical pump, graphene undergoes ultrafast heating (
In our calculations, we only use the possible temperature range of graphene electrons to calculate temperature-dependent conductivity. Strictly, in this case, the ultrafast pump-induced effects of graphene should be considered in the analysis. However, we notice that some works already get several beautiful results of that, by which the temperature and graphene conductivity can be calculated in a consistent manner [35,45]. On the other hand, in this work, we only hope to theoretically reveal the possibility of this ultrafast pump-induced OTT of CR in the GHMs. The calculation and analysis of the entire ultrafast pump-induced processes in graphene are beyond the scope of this work.
The graphene conductivity considering photothermal effects can be calculated as [35,45]
According to these formulas, the imaginary part of the temperature-dependent graphene conductivity,
Figure 7.Temperature-dependent photothermal properties. (a) Imaginary part of temperature-dependent conductivity,
Concretely, for example, consider a CR frequency of 30 THz. When
We have demonstrated that the macroscopic EMT and microscopic SMT could unveil basic properties of effective CR and plasmon modes excited by low-energy electrons in the GHM, in which the electron velocity for excitation can be
For demonstration, we use the widely used nonlocal graphene conductivity model based on random-phase approximation with relaxation-time approximation, which disregards the many-body interactions between electrons [47]. The imaginary part of the nonlocal graphene conductivity,
Figure 8.Nonlocal properties. (a) Imaginary part of nonlocal graphene conductivity,
Then, the real part of the nonlocal effective in-plane permittivity,
4. CONCLUSION AND OUTLOOK
In this work, we comprehensively studied the OTT of CR in the GHMs theoretically for the first time. We propose that the topological phase or the OTT frequency of the GHM determines the feasibility of CR generation by low-energy electrons. These parameters can be modified by various factors in regions such as the graphene and dielectric parameters. They can also be manipulated by an external optical pump in an ultrafast manner. In the GHMs, hyperbolic CR, also known as threshold-free CR, is produced by the superposition of plasmon modes excited in different graphene layers effectively. Both the topological state and electron velocity should be adjusted to ensure CR generation. Combining the advantages of the EMT and SMT, we can clarify the detailed mechanisms of the OTT of CR in the GHMs from both macroscopic and microscopic perspectives. Moreover, the nonlocality and ultrafast pump-induced photothermal properties of CR in hyperbolic media are also important and should be considered in future work.
Nevertheless, our results are achieved without considering the effects of the many-body interactions in graphene, and the GHM is only one of the hyperbolic media, both of which should be extended to satisfy more complex and broader situations in the future. Specifically, the Fermi velocity of graphene is treated as a constant value throughout this work. However, the Fermi velocity of graphene is just the nonlocality-induced lower velocity threshold of CR in the GHMs. As far as we know, the Fermi velocity of graphene can be tuned by the electron-electron interactions [49]. By this method, the Fermi velocity of graphene can be promoted near threefold (
For the experimental demonstration in the future, it requires two main technical conditions as follows: first, the effective fabrication and electrical tuning of high-quality graphene and the GHMs; second, the efficient production and flexible controlling of the free electrons, and the precise electromagnetic radiation measurements. For the former, previous experimental results indicate that the fabrication and manipulation of the GHMs in the mid-infrared are achievable and realizable [41,42]. For the latter, previous experimental results indicate the feasibility of the observation of the interactions between HMMs and free electrons [17,22]. Additionally, in this work, we also propose an ultrafast CR device caused by the ultrafast photothermal effects of graphene. In recent research, experimental achievements in the fields of ultrafast lasers and ultrafast electron pulses can provide high-performance measurements [1–5]. With the developments in ultrafast lasers and ultrafast electron pulses, we believe our designs can be realized in the future.
Although the OTT of CR in the GHMs is well investigated in this work, the properties and applications of OTT can hardly cover. Specifically, the OTT can make wave propagation in the forbidden directions of the optical systems [56], produce the optical spin–Hall effect [57], and so on. For the optical devices based on OTT, these include the hyper-lens [58], the epsilon-near-zero devices [59], and the solar cell for energy transfer [60]. With the development of topological photonics, the effects and devices based on the OTT can be achieved in the future [32–34].
On the other hand, in the terahertz and mid-infrared bands, many optical phenomena associated with the OTT can be achieved in materials and metamaterials, and these materials and metamaterials can be controlled by electronic, magnetic, and thermionic methods [61–63]. We believed that these materials and metamaterials that possess brilliant properties can be combined with free electrons in the future and can provide more possibilities in the research of the terahertz and mid-infrared sources, devices, and applications.
The interaction between free electrons and materials or metamaterials is expected to gain importance in future research and applications. In addition to graphene, various natural materials can be used as platforms for studying free-electron-based light–matter interactions, such as hexagonal boron nitride, transition metal dichalcogenides, topological materials, and other polariton materials [64]. The ultrafast property of electrons will be another hotspot for studying and using light–matter interactions based on intense ultrafast laser and free-electron sources [1–3]. Efficient, compact, low-cost, and highly tunable stimulated-CR and CR-based free-electron laser sources, detectors, and electronic/photonic devices are challenging to develop yet promising.
We expect that the mechanisms, explanations, and findings of this study will support future work in the field. Free-electron-based sciences, technologies, and applications will continue expanding, and we believe that our work is valuable for understanding complex interactions between free electrons and novel materials/metamaterials. Furthermore, our findings may pave the way for the development of future high-performance integrated and tunable free-electron-based light sources, devices, and applications.
References
[1] F. J. García De Abajo. Optical excitations in electron microscopy. Rev. Mod. Phys., 82, 209(2010).
[2] A. Polman, M. Kociak, F. J. García de Abajo. Electron-beam spectroscopy for nanophotonics. Nat. Mater., 18, 1158-1171(2019).
[3] F. J. García De Abajo, V. Di Giulio. Optical excitations with electron beams: challenges and opportunities. ACS Photon., 8, 945-974(2021).
[4] L. J. Wong, I. Kaminer, O. Ilic, J. D. Joannopoulos, M. Soljačić. Towards graphene plasmon-based free-electron infrared to X-ray sources. Nat. Photonics, 10, 46-52(2016).
[5] M. Shentcis, A. K. Budniak, X. Shi, R. Dahan, Y. Kurman, M. Kalina, H. Herzig Sheinfux, M. Blei, M. K. Svendsen, Y. Amouyal, S. Tongay, K. S. Thygesen, F. H. L. Koppens, E. Lifshitz, F. J. García de Abajo, L. J. Wong, I. Kaminer. Tunable free-electron X-ray radiation from van der Waals materials. Nat. Photonics, 14, 686-692(2020).
[6] P. A. Cherenkov. Radiation from high-speed particles. Science, 131, 136-142(1960).
[7] I. E. Tamm. General characteristics of Vavilov-Cherenkov radiation. Science, 131, 206-210(1960).
[8] I. M. Frank. Optics of light sources moving in refractive media. Science, 131, 702-712(1960).
[9] V. L. Ginzburg. Transition radiation and transition scattering. Phys. Scr., 1982, 182(1982).
[10] I. Frank, I. Tamm. Radiation by uniformly moving sources (Vavilov–Cherenkov effect, transition radiation, and other phenomena). Physics-Uspekhi, 39, 973-982(1996).
[11] S. J. Smith, E. M. Purcell. Visible light from localized surface charges moving across a grating. Phys. Rev., 92, 1069(1953).
[12] Z. Su, B. Xiong, Y. Xu, Z. Cai, J. Yin, R. Peng, Y. Liu. Manipulating Cherenkov radiation and Smith–Purcell radiation by artificial structures. Adv. Opt. Mater., 7, 1801666(2019).
[13] P. Cherenkov. Visible glow of pure liquids under the action of γ-radiation. Dokl. Akad. Nauk SSSR, 2, 451(1934).
[14] P. A. Cherenkov. Visible radiation produced by electrons moving in a medium with velocities exceeding that of light. Phys. Rev., 52, 378-379(1937).
[15] I. E. Tamm, I. M. Frank. Coherent visible radiation of fast electrons passing through matter. Dokl. Akad. Nauk SSSR, 14, 107-112(1937).
[16] M. Günay, Y. L. Chuang, M. E. Tasgin. Continuously-tunable Cherenkov-radiation-based detectors via plasmon index control. Nanophotonics, 9, 1479-1489(2020).
[17] X. Lin, H. Hu, S. Easo, Y. Yang, Y. Shen, K. Yin, M. P. Blago, I. Kaminer, B. Zhang, H. Chen, J. Joannopoulos, M. Soljačić, Y. Luo. A Brewster route to Cherenkov detectors. Nat. Commun., 12, 5554(2021).
[18] Y. Gong, Q. Zhou, M. Hu, Y. Zhang, X. Li, H. Gong, J. Wang, D. Liu, Y. Liu, Z. Duan, J. Feng. Some advances in theory and experiment of high-frequency vacuum electron devices in China. IEEE Trans. Plasma Sci., 47, 1971-1990(2019).
[19] T. M. Shaffer, E. C. Pratt, J. Grimm. Utilizing the power of Cerenkov light with nanotechnology. Nat. Nanotechnol., 12, 106-117(2017).
[20] S. Liu, P. Zhang, W. Liu, S. Gong, R. Zhong, Y. Zhang, M. Hu. Surface polariton Cherenkov light radiation source. Phys. Rev. Lett., 109, 153902(2012).
[21] M. Silveirinha. A low-energy Cherenkov glow. Nat. Photonics, 11, 269-271(2017).
[22] F. Liu, L. Xiao, Y. Ye, M. Wang, K. Cui, X. Feng, W. Zhang, Y. Huang. Integrated Cherenkov radiation emitter eliminating the electron velocity threshold. Nat. Photonics, 11, 289-292(2017).
[23] P. Shekhar, S. Pendharker, H. Sahasrabudhe, D. Vick, M. Malac, R. Rahman, Z. Jacob. Extreme ultraviolet plasmonics and Cherenkov radiation in silicon. Optica, 5, 1590-1596(2018).
[24] A. A. Govyadinov, A. Konečná, A. Chuvilin, S. Vélez, I. Dolado, A. Y. Nikitin, S. Lopatin, F. Casanova, L. E. Hueso, J. Aizpurua, R. Hillenbrand. Probing low-energy hyperbolic polaritons in van der Waals crystals with an electron microscope. Nat. Commun., 8, 95(2017).
[25] T. Qu, F. Liu, Y. Lin, K. Cui, X. Feng, W. Zhang, Y. Huang. Cherenkov radiation generated in hexagonal boron nitride using extremely low-energy electrons. Nanophotonics, 9, 1491-1499(2020).
[26] C. Maciel-Escudero, A. Konečná, R. Hillenbrand, J. Aizpurua. Probing and steering bulk and surface phonon polaritons in uniaxial materials using fast electrons: hexagonal boron nitride. Phys. Rev. B, 102, 115431(2020).
[27] C. Luo, M. Ibanescu, S. G. Johnson, J. D. Joannopoulos. Cerenkov radiation in photonic crystals. Science, 299, 368-371(2003).
[28] S. Xi, H. Chen, T. Jiang, L. Ran, J. Huangfu, B. I. Wu, J. A. Kong, M. Chen. Experimental verification of reversed Cherenkov radiation in left-handed metamaterial. Phys. Rev. Lett., 103, 194801(2009).
[29] Z. Duan, X. Tang, Z. Wang, Y. Zhang, X. Chen, M. Chen, Y. Gong. Observation of the reversed Cherenkov radiation. Nat. Commun., 8, 14901(2017).
[30] S. Gong, M. Hu, Z. Wu, H. Pan, H. Wang, K. Zhang, R. Zhong, J. Zhou, T. Zhao, D. Liu, W. Wang, C. Zhang, S. Liu. Direction controllable inverse transition radiation from the spatial dispersion in a graphene-dielectric stack. Photon. Res., 7, 1154-1160(2019).
[31] X. Zhang, M. Hu, Z. Zhang, Y. Wang, T. Zhang, X. Xu, T. Zhao, Z. Wu, R. Zhong, D. Liu, Y. Wei, Y. Gong, S. Liu. High-efficiency threshold-less Cherenkov radiation generation by a graphene hyperbolic grating in the terahertz band. Carbon, 183, 225-231(2021).
[32] L. Lu, J. D. Joannopoulos, M. Soljačić. Topological photonics. Nat. Photonics, 8, 821-829(2014).
[33] T. Ozawa, H. M. Price, A. Amo, N. Goldman, M. Hafezi, L. Lu, M. C. Rechtsman, D. Schuster, J. Simon, O. Zilberberg, I. Carusotto. Topological photonics. Rev. Mod. Phys., 91, 015006(2019).
[34] H. N. S. Krishnamoorthy, Z. Jacob, E. Narimanov, I. Kretzschmar, V. M. Menon. Topological transitions in metamaterials. Science, 336, 205-209(2012).
[35] R. Yu, R. Alaee, R. W. Boyd, F. J. G. De Abajo. Ultrafast topological engineering in metamaterials. Phys. Rev. Lett., 125, 037403(2020).
[36] A. J. Sternbach, S. H. Chae, S. Latini, A. A. Rikhter, Y. Shao, B. Li, D. Rhodes, B. Kim, P. J. Schuck, X. Xu, X.-Y. Zhu, R. D. Averitt, J. Hone, M. M. Fogler, A. Rubio, D. N. Basov. Programmable hyperbolic polaritons in van der Waals semiconductors. Science, 371, 617-620(2021).
[37] A. N. Grigorenko, M. Polini, K. S. Novoselov. Graphene plasmonics. Nat. Photonics, 6, 749-758(2012).
[38] G. X. Ni, A. S. McLeod, Z. Sun, L. Wang, L. Xiong, K. W. Post, S. S. Sunku, B. Y. Jiang, J. Hone, C. R. Dean, M. M. Fogler, D. N. Basov. Fundamental limits to graphene plasmonics. Nature, 557, 530-533(2018).
[39] F. H. L. Koppens, D. E. Chang, F. J. García De Abajo. Graphene plasmonics: a platform for strong light–matter interactions. Nano Lett., 11, 3370-3377(2011).
[40] A. Poddubny, I. Iorsh, P. Belov, Y. Kivshar. Hyperbolic metamaterials. Nat. Photonics, 7, 948-957(2013).
[41] Y. C. Chang, C. H. Liu, C. H. Liu, S. Zhang, S. R. Marder, E. E. Narimanov, Z. Zhong, T. B. Norris. Realization of mid-infrared graphene hyperbolic metamaterials. Nat. Commun., 7, 10568(2016).
[42] H. Lin, B. C. P. Sturmberg, K. Te Lin, Y. Yang, X. Zheng, T. K. Chong, C. M. de Sterke, B. Jia. A 90-nm-thick graphene metamaterial for strong and extremely broadband absorption of unpolarized light. Nat. Photonics, 13, 270-276(2019).
[43] J. Benedicto, R. Pollès, C. Ciracì, E. Centeno, D. R. Smith, A. Moreau. Numerical tool to take nonlocal effects into account in metallo-dielectric multilayers. J. Opt. Soc. Am. A, 32, 1581-1588(2015).
[44] H. Hu, X. Lin, J. Zhang, D. Liu, P. Genevet, B. Zhang, Y. Luo. Nonlocality induced Cherenkov threshold. Laser Photon. Rev., 14, 2000149(2020).
[45] R. Yu, Q. Guo, F. Xia, F. J. G. de Abajo. Photothermal engineering of graphene plasmons. Phys. Rev. Lett., 121, 057404(2018).
[46] H. Hu, D. Gao, X. Lin, S. Hou, B. Zhang, Q. J. Wang, Y. Luo. Directing Cherenkov photons with spatial nonlocality. Nanophotonics, 9, 3435-3442(2020).
[47] E. Galiffi, P. A. Huidobro, P. A. D. Gonçalves, N. A. Mortensen, J. B. Pendry. Probing graphene’s nonlocality with singular metasurfaces. Nanophotonics, 9, 309-316(2020).
[48] S. Raza, S. I. Bozhevolnyi, M. Wubs, N. A. Mortensen. Nonlocal optical response in metallic nanostructures. J. Phys. Condens. Matter, 27, 183204(2015).
[49] D. C. Elias, R. V. Gorbachev, A. S. Mayorov, S. V. Morozov, A. A. Zhukov, P. Blake, L. A. Ponomarenko, I. V. Grigorieva, K. S. Novoselov, F. Guinea, A. K. Geim. Dirac cones reshaped by interaction effects in suspended grapheme. Nat. Phys., 7, 701-704(2011).
[50] C. Hwang, D. A. Siegel, S. K. Mo, W. Regan, A. Ismach, Y. Zhang, A. Zettl, A. Lanzara. Fermi velocity engineering in graphene by substrate modification. Sci. Rep., 2, 590(2012).
[51] D. A. Siegel, W. Regan, A. V. Fedorov, A. Zettl, A. Lanzara. Charge-carrier screening in single-layer grapheme. Phys. Rev. Lett., 110, 146802(2013).
[52] T. Stauber, P. Parida, M. Trushin, M. V. Ulybyshev, D. L. Boyda, J. Schliemann. Fermi velocity renormalization and optical response. Phys. Rev. Lett., 118, 266801(2017).
[53] O. Vafek, J. Kang. Hidden symmetry in twisted bilayer graphene with Coulomb interactions. Phys. Rev. Lett., 125, 257602(2020).
[54] C. H. Park, F. Giustino, M. L. Cohen, S. G. Louie. Velocity renormalization and carrier lifetime in graphene from the electron-phonon interaction. Phys. Rev. Lett., 99, 086804(2007).
[55] F. Escudero, J. S. Ardenghi. Fermi velocity reduction in graphene due to enhanced vacuum fluctuations. J. Phys. Condens. Matter, 33, 485502(2021).
[56] J. Duan, G. Álvarez-Pérez, K. V. Voronin, I. Prieto, J. Taboada-Gutiérrez, V. S. Volkov, J. Martín-Sánchez, A. Y. Nikitin, P. Alonso-González. Enabling propagation of anisotropic polaritons along forbidden directions via a topological transition. Sci. Adv., 7, eabf2690(2021).
[57] W. Wu, W. Wu, S. Chen, W. Xu, Z. Liu, R. Lou, L. Shen, H. Luo, H. Luo, S. Wen, X. Yin, X. Yin, X. Yin. Weak-value amplification for the optical signature of topological phase transitions. Photon. Res., 8, B47-B54(2020).
[58] Y. Gelkop, F. Di Mei, S. Frishman, Y. Garcia, L. Falsi, G. Perepelitsa, C. Conti, E. DelRe, A. J. Agranat. Hyperbolic optics and superlensing in room-temperature KTN from self-induced k-space topological transitions. Nat. Commun., 12, 7241(2021).
[59] P. N. Dyachenko, S. Molesky, A. Y. Petrov, M. Störmer, T. Krekeler, S. Lang, M. Ritter, Z. Jacob, M. Eich. Controlling thermal emission with refractory epsilon-near-zero metamaterials via topological transitions. Nat. Commun., 7, 11809(2016).
[60] R. Deshmukh, S. A. Biehs, E. Khwaja, T. Galfsky, G. S. Agarwal, V. M. Menon. Long-range resonant energy transfer using optical topological transitions in metamaterials. ACS Photonics, 5, 2737-2741(2018).
[61] Y. Xiang, X. Dai, J. Guo, H. Zhang, S. Wen, D. Tang. Critical coupling with graphene-based hyperbolic metamaterials. Sci. Rep., 4, 5483(2014).
[62] T. T. Lv, Y. X. Li, H. F. Ma, Z. Zhu, Z. P. Li, C. Y. Guan, J. H. Shi, H. Zhang, T. J. Cui. Hybrid metamaterial switching for manipulating chirality based on VO2 phase transition. Sci. Rep., 6, 23186(2016).
[63] J. Shi, Z. Li, D. K. Sang, Y. Xiang, J. Li, S. Zhang, H. Zhang. THz photonics in two dimensional materials and metamaterials: properties, devices and prospects. J. Mater. Chem. C, 6, 1291-1306(2018).
[64] D. N. Basov, M. M. Fogler, F. J. García De Abajo. Polaritons in van der Waals materials. Science, 354, aag1992(2016).
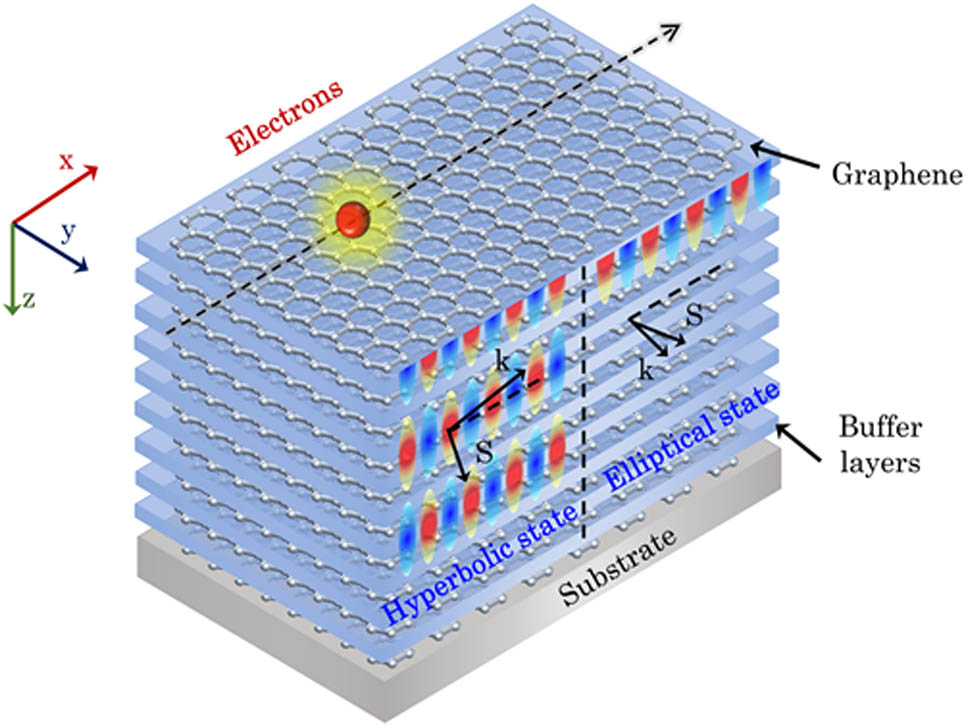
Set citation alerts for the article
Please enter your email address