Fig. 1. Conceptual schematic of the dual-spin/frequency multiplexing metasurface for spin-to-OAM conversion and its inverse design approach. Light with opposite spin states and different frequencies converted to optic vortex carrying arbitrary OAM mode after being reflected from the metasurface. The inset shows the spiral phase distribution of the four VBs with topological charges , and . When the wavefronts for quadruplex channels are input to the deep-learning-assisted methodology, physical parameters for all the meta-atoms of the metasurface can be automatically output without human interference. are the physical parameters of the meta-atom mainly determining its EM responses in quadruplex channels.
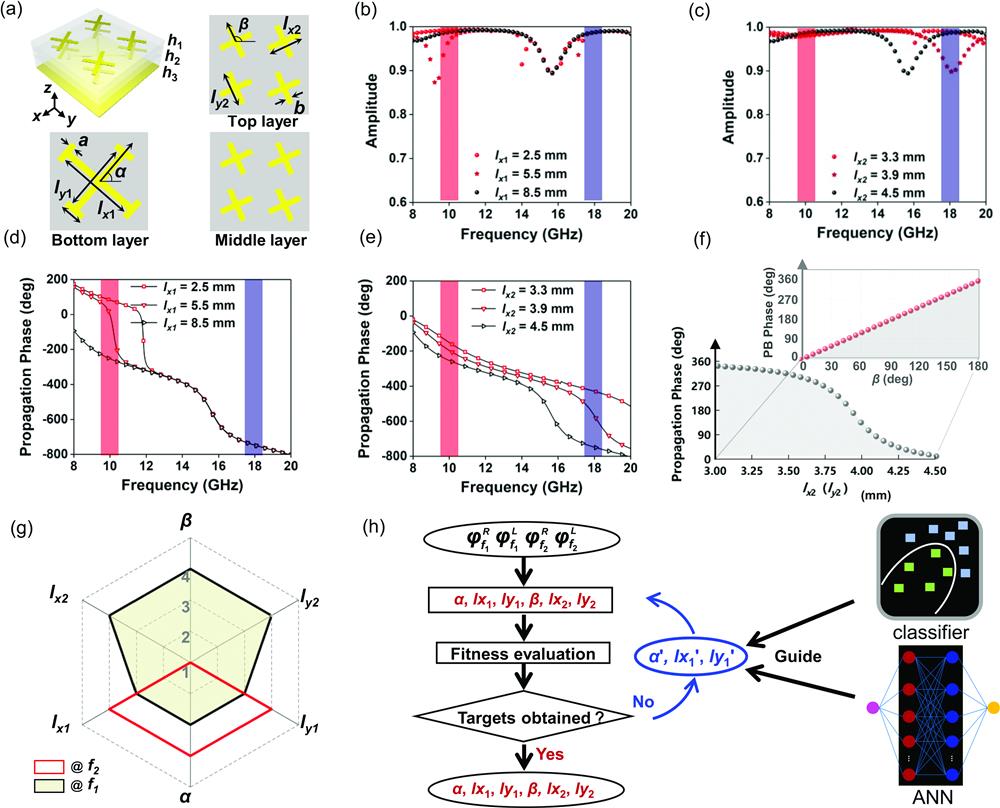
Fig. 2. EM response of the meta-atom and realization of the inverse design. (a) Schematic of the meta-atom with multilayer configuration. The geometric parameters of the top layer and middle one are the same. When rotation angles of all the cross resonators remain 0 deg, the simulated linearly copolarized amplitude under -LP illumination with (b) only varying from 2.5 to 8.5 mm or (c) only varying from 3.3 to 4.5 mm. (d) and (e) The simulated phase spectra corresponding to (b) and (c), respectively. In particular, parameters are fixed at {6 mm, 4.5 mm, 3.3 mm} when varies, and parameters are fixed at {4.5 mm, 8 mm, 6 mm} when varies. (f) At high frequency, simulated geometric phase response with the rotation angle varying from to 90 deg and the propagation phase response with different dimensions at 17.8 GHz. The parameters , , and are fixed at 4.5, 8, and 6 mm, respectively. Actually, the changes in these three parameters hardly affect the propagation phase for -LP at high frequency. For the geometric phase curve at high frequency, the fixed values of and are the same as above, and , are fixed to 4.5 and 3.93 mm, aiming to ensure a phase difference of 180 deg between two orthogonal LP waves. (g) Influence of six parameters on wavefront modulation in the dual frequency. (h) Flow chart of the adaptive optimization process guided by the deep-learning methods.
Fig. 3. Realization of dual-frequency spin-to-orbit metaconverter. (a) Physical parameter distributions of the elements in sample 1. (b) Calculated phase distribution for RCP channel at 9.8 GHz, LCP channel at 9.8 GHz, RCP channel at 17.8 GHz, and LCP channel at 17.8 GHz. (c) Photograph of the fabricated spin-to-orbit metaconverter. (d) Normalized simulated power patterns in momentum space. (e) Simulated and measured intensity patterns and phase patterns of different OAM beams in the four channels.
Fig. 4. Realization of dual-frequency VVB metamultiplexer. (a) Parameter distributions of the elements in sample 2. (b) Calculated phase distributions for RCP channel at 9.8 GHz, LCP channel at 9.8 GHz, RCP channel at 17.8 GHz, and LCP channel at 17.8 GHz. (c) Photograph of the fabricated VVB meta-multiplexer. (d) Normalized simulated power patterns and their positions on the three HOPSs. Beam #1 is designed at 9.8 GHz and beams #2 and #3 are designed at 17.8 GHz. (e) Simulated and measured intensity patterns of two orthogonal LP components. The white arrows indicate the detected polarization direction.