
- Journal of Semiconductors
- Vol. 40, Issue 4, 042901 (2019)
Abstract
1. Introduction
Quantum structures in low-dimensional materials (LDM) have been employed powerfully in the last twenty years due to their fundamental physical properties[
Recently, using a Gaussian potential, many researchers have studied the electronic and polaronic properties. For example, Nandi et al.[
Based on these investigations, we found that a new confining potential is proposed as the Gaussian potential. The new potential, which contains AGCP, is practical for calculating GS energy and the GS binding energy. However, considering AGCP to study the VF, the GS energy and the GS binding energy isn’t investigated.
In the present article, we use simple methods (linear combination operator and twice unitary transformation) to investigate the polaron’s VF, GS energy and GS binding energy in the AGCP QWs. Our results provide a theoretical basis for low dimensional nanomaterials.
2. Theory model
An electron is confined in the QW with AGCP. The electron is moving in a GaAs semiconductor crystal and interacting with bulk longitudinal optical (LO) phonons. The system Hamiltonian has the following forms:
where
In the above equation, m is the electron band mass,
We operate the twice unitary transformations to the Hamiltonian in Eq. (1)[
where
where
The variation of
where
3. Results and discussions
The effective mass of GaAs is taken to be a value of 0.0675m0, the strength of electron-LO-phonon coupling is set to 0.068, and the phonon energy is
Fig. 1 depicts the VF of the polaron changing with the barrier height of the QW
Figure 3.Relational curves of the GS binding energy
In Fig. 3, we illustrate the GS binding energy varying with the barrier height of the QW and the range of the AGCP. The four lines correspond to the barrier height of the QW:
From Figs. 1, 2 and 3 one can easily find that the VF decreases with the range of the AGCP, whereas the absolute value of GS energy and the GS binding energy increase with it because the electron motion is confined by the AGCP. The decrease of the confinement potential and z results in the reduction of the particle motion range, the electron energy and the interaction of the electron with the phonons around it are enhanced. So, the VF is increased. These physics properties contribute to the interesting quantum confining effect.
However, from the first term in Eq. (9), due to the range of the AGCP term, the GS energy is a positive value, whereas the total GS energy is negative. Moreover, from the last term in Eq. (10), due to the range of the AGCP term, the GS binding energy is a negative value. It is clear that the absolute values of GS energy and the GS binding energy increase with range of the AGCP. Here we can find that the VF, the GS energy and the GS binding energy in the QW can be adjusted by changing the barrier height of the QW and the range of the AGCP .
In summary, it is well known that the barrier height of the QW and the range of AGCP in the growth direction of the QW are vital factors for polaron research in the QW with AGCP.
The asymmetric Gaussian type QW is a new type of low-dimensional nano-quantum system. Due to its special properties, this new structure has attracted the attention of many scholars. This structure is characterized by the existence of a limited potential in the direction of growth
Through the above discussions, we find that the QW barrier height and the range of Gaussian confinement potential in the growth direction of the QW are important physical quantities for studying the properties of the energy-level and vibrational frequency of weak -coupling polarons in a QW with AGCP.
4. Conclusions
In conclusions, the VF, the GS energy and the GS binding energy of the polaron weakly-coupled in the QW with AGCP are investigated by the above-mentioned methods. They have the following properties: (1) The VF, the absolute value of GS energy and the GS binding energy will increase with the barrier height of the QW. (2) The VF will decrease with the range of the AGCP in the QW, whereas the absolute value of GS energy and the GS binding energy increase with it. Here we can find that changing the barrier height of the QW and the range of the AGCP can adjust the three physics quantities in the special QW.
Acknowledgement
This work was supported by the National Science Foundation of China (Nos. 11464033, 11464034).
References
[1] O Ozturk, E Ozturk, S Elagoz. The effect of barrier width on the electronic properties of double GaAlAs/GaAs and GaInAs/GaAs quantum wells. J Mol Struct, 1156, 726(2018).
[2] P S Samokhvalov, P A Linkov, M A Zvaigzne et al. Optical properties of core-multishell quantum dots. KnE Energy Phys, 3, 449(2018).
[3] Z Cai, B Liu, X Zou et al. Chemical vapor deposition growth and applications of two-dimensional materials and their heterostructures. Chem Rev, 118, 6091(2018).
[4] M Belitsch, C Gruber, H Ditlbacher et al. Gray state dynamics in the blinking of single type I colloidal quantum dots. Nano, 13, 1850039(2018).
[5] J Müßener, A T Ludwig, S Kalinowski et al. Optical emission of GaN/AlN quantum-wires–the role of charge transfer from a nanowire template. Nanoscale, 10, 5591(2018).
[6] L J Chen. Synthesis and optical properties of lead-free cesium germanium halide perovskite quantum rods. RSC Adv, 8, 18396(2018).
[7] P Moon, C Forsythe, X Zhou et al. Electronic structure engineering of graphene using patterned dielectric superlattices. Bull Am Phys Soc(2018).
[8] X Wu, J Li, M Briggeman et al. Breaking electron pairs in pseudogap state in SmTiO3/SrTiO3/SmTiO3 quantum wells. Bull Am Phys Soc(2018).
[9] O Ambacher, J Majewski, C Miskys et al. Pyroelectric properties of Al (In) GaN/GaN hetero-and quantum well structures. J Phys: Conden Matter, 14, 3399(2002).
[10] T Makino, Y Segawa, M Kawasaki et al. Optical properties of excitons in ZnO-based quantum well heterostructures. Semicond Sci Technol, 20, S78(2005).
[11] M E Aumer, S F Leboeuf, S M Bedair et al. Effects of tensile and compressive strain on the luminescence properties of AlInGaN/InGaN quantum well structures. Appl Phys Lett, 77, 821(2000).
[12] S Nandi. The quantum Gaussian well. Am J Phys, 78, 1341(2010).
[13] J Wu, K Guo, G Liu. Polaron effects on nonlinear optical rectification in asymmetrical Gaussian potential quantum wells with applied electric fields. Physica B, 446, 59(2014).
[14] J L Xiao. The effects of hydrogen-like impurity and temperature on state energies and transition frequency of strong-coupling bound polaron in an asymmetric gaussian potential quantum well. J Low Temp Phys, 192, 41(2018).
[15] J L Xiao. Properties of strong-coupling polaron in an asymmetrical gaussian confinement potential quantum well. Journal of Inner Mongolia University for Nationalities, 30, 369(2015).
[16] T D Lee, F E Low, D Pines. The motion of slow electrons in a polar crystal. Phys Rev, 90, 297(1953).
[17] W J Huybrechts. Note on the ground-state energy of the Feynman polaron model. J Physs C, 9, L211(1976).
[18]
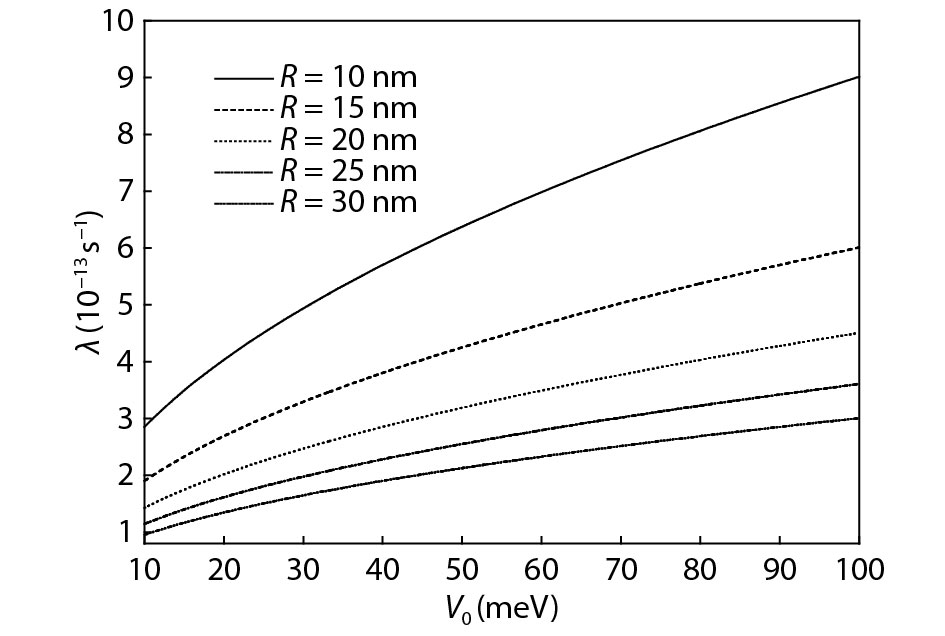
Set citation alerts for the article
Please enter your email address