
- Photonics Research
- Vol. 10, Issue 7, 1695 (2022)
Abstract
1. INTRODUCTION
The spin of a photon is the quantum mechanical counterpart of the polarization of light, and each photon of left-/right-handed circular polarized (LHCP/RHCP) light carries a spin angular momentum of
The building element of SOI metasurfaces is generally called a Pancharatnam–Berry optical element (PBOE) [13,22]. A perfect PBOE will completely flip the input circular polarization to its orthogonal state with an extra geometric phase shift that is double the element’s orientation angle. Single layer plasmonic metasurfaces were first utilized to study SOI [16,23]. However, their power efficiency was low due to the compromise between anisotropy and transmittance [23]. To increase the SOI efficiency, multilayer plasmonic, coherently controlled, and dielectric metasurfaces have been adopted [22,24,25]. Specifically, dielectric metasurfaces consisting of high contrast dielectric elements have recently attracted increasing research interest [26,27]. For such a dielectric element, the anisotropy is flexibly tailorable over a large range, and the material loss can be made negligible by properly selecting dielectric materials, endowing it with a significant advantage in the search for a perfect PBOE.
Besides the power efficiency, another challenge of SOI is that the inherent geometric phase shifts of a PBOE over different circularly polarized inputs are equal in magnitude with opposite signs [16]. Therefore, the achieved functionalities are limited only through the geometric phase. To make the imposed phase shifts for RHCP and LHCP inputs independent and thus the spin-control more flexible, further transport phases have been introduced by utilizing PBOEs of various geometries [28]. Through the combination of the transport phase and the geometric phase, independent and complete phase and wavefront control over orthogonal circular polarizations has been demonstrated. Such a method has greatly expanded the applications of PBOEs in polarization optics [29–31], including polarization detection [32], splitting [33], polarization contrast imaging [34], multiplexing, and encryption [35–37].
Sign up for Photonics Research TOC. Get the latest issue of Photonics Research delivered right to you!Sign up now
In this context, we present a design method of spin-dependent all-silicon dielectric metasurfaces by simultaneously involving the geometric phase and transport phase in the constituting PBOEs. The PBOEs are anisotropic rectangular posts distributed in a tetragonal lattice on silicon substrate. Two functional metasurface devices are designed and experimentally characterized in the terahertz regime, including a spin-selective Bessel/half-wave metasurface device and a spin-dependent Bessel/vortex beam generator. The Bessel/half-wave metasurface generates a cross-polarized Bessel beam under the LHCP incidence, whereas it only flips the polarization under the RHCP incidence. The Bessel/vortex beam generator produces Bessel and vortex beams separately under the LHCP and RHCP incidences. Such metasurface devices have the potential to help realize miniature THz systems, with key applications in spin switching, contrast imaging, coding, and encryption.
2. DESIGN STRATEGY
A perfect PBOE functions as a half-wave plate, i.e., there is a 180° phase difference between the phase shifts along the local fast and slow axes of the PBOE. The corresponding rotated transmission matrix in the Cartesian coordinate system can be expressed as [33]
Figure 1.(a) Schematic of the spin-dependent metasurface, which consists of PBOEs and can generate two arbitrarily designable beam functions,
To further investigate the phase control and cross-polarization conversion performances of the 8 fundamental PBOEs, the electric field distributions at 1.0 THz are simulated, as shown in Fig. 2(a). It can be seen that the output phases of
Figure 2.(a) Simulated electric field distributions of
3. RESULTS AND DISCUSSION
To experimentally verify that the selected PBOEs meet the two criteria, i.e., perfect cross-polarization conversion and complete phase coverage, a polarization conversion deflector (PCD) is first constructed by patterning the PBOEs into a linear phase distribution along the
Figure 3.(a) Microscopic image of the fabricated PCD. Scale bar: 300 μm. (b) and (c) The measured normalized intensity distributions as a function of the diffraction angle and the frequency of the
The PCD was then characterized using our customized all-fiber terahertz time-domain spectrometer (TDS) system [38]. For bandwidth characterization and simplicity, a linearly polarized input is used here. Figures 3(b) and 3(c) show the measured
From the measured results of the PCD, it can be concluded that the selected basic PBOEs can well satisfy our requirements in achieving efficient and independent control over the complete phases of the orthogonal circular polarizations at 1.1 THz. Following the above experimental confirmation, we further fabricate two functional devices.
One is a Bessel/half-wave metasurface, which serves as a Bessel generator under the LHCP incidence but as a half-wave plate under the RHCP incidence. To generate the Bessel beam under the LHCP incidence, a linear gradient of
Meanwhile, attributing to the inherent cross-polarization conversion function of the PBOE metasurface,
Figure 4.(a) Microscopic image of a fraction of the fabricated Bessel/half-wave metasurface. Scale bar: 300 μm. (b)–(d) The measured normalized RHCP intensity distributions at the longitudinal propagation plane at
The second device is a Bessel/vortex metasurface, which is capable of producing Bessel and vortex beams separately by switching between the LHCP and RHCP incidences. The Bessel beam for the LHCP input is designed to the same specifications as the former device, while the first-order vortex beam for the RHCP input is designed with a topological charge
Figure 5.(a) Microscopic image of a fraction of the fabricated Bessel/vortex metasurface. Scale bar: 300 μm. (b)–(d) The measured normalized RHCP intensity distributions at the longitudinal propagation plane at
4. CONCLUSION
Terahertz all-silicon dielectric metasurfaces for spin-dependent and complete wavefront control are realized using PBOEs through introducing extra geometry inhomogeneity besides the rotation inhomogeneity. In this work, a simplified design strategy is utilized to facilitate the metasurface design attributing to the structure symmetry. Based on the simplified design, several exemplar metasurfaces are accomplished using the minimized fundamental constituting elements. One is used to confirm the performance of the selected PBOEs, while the other two metasurfaces demonstrate functional devices—a Bessel/half-wave metasurface and a Bessel/vortex metasurface. The measured results agree well with our design. Such dielectric metasurfaces are easy to fabricate, compatible with semiconductor integration, and have promising applications in ultra-compact and spin-involved optical terahertz imaging system and communication systems for polarization contrast imaging, coding, and encryption.
APPENDIX A: SIMULATION METHODS
To select the proper PBOEs, CST Microwave Studio is used to simulate the transmissions (including amplitude and shift) of the silicon posts, in which we set
APPENDIX B: SAMPLE FABRICATION
The silicon dielectric metasurfaces were fabricated using conventional photolithography and deep reactive ion etching (DRIE) [
References
[1] A. I. Akhiezer, V. B. Berestetskii. Quantum Electrodynamics(1965).
[2] J. Allen, S. M. Barnett, M. J. Padgett. Optical Angular Momentum(2003).
[3] A. T. O’Neil, I. MacVicar, L. Allen, M. J. Padgett. Intrinsic and extrinsic nature of the orbital angular momentum of a light beam. Phys. Rev. Lett., 88, 053601(2002).
[4] K. Y. Bliokh, F. J. Rodríguez-Fortuño, F. Nori, A. V. Zayats. Spin-orbit interactions of light. Nat. Photonics, 9, 796-808(2015).
[5] M. Onoda, S. Murakami, N. Nagaosa. Hall effect of light. Phys. Rev. Lett., 93, 083901(2004).
[6] O. Hosten, P. Kwiat. Observation of the spin Hall effect of light via weak measurements. Science, 319, 787-790(2008).
[7] K. Y. Bliokh, A. Niv, V. Kleiner, E. Hasman. Geometrodynamics of spinning light. Nat. Photonics, 2, 748-753(2008).
[8] S.-Y. Lee, I.-M. Lee, J. Park, S. Oh, W. Lee, K.-Y. Kim, B. Lee. Role of magnetic induction currents in nanoslit excitation of surface plasmon polaritons. Phys. Rev. Lett., 108, 213907(2012).
[9] N. Shitrit, I. Yulevich, E. Maguid, D. Ozeri, D. Veksler, V. Kleiner, E. Hasman. Spin-optical metamaterial route to spin-controlled photonics. Science, 340, 724-726(2013).
[10] J. Lin, J. P. B. Mueller, Q. Wang, G. Yuan, N. Antoniou, X.-C. Yuan, F. Capasso. Polarization-controlled tunable directional coupling of surface plasmon polaritons. Science, 340, 331-334(2013).
[11] F. J. Rodríguez-Fortuño, I. Barber-Sanz, D. Puerto, A. Griol, A. Martínez. Resolving light handedness with an on-chip silicon microdisk. ACS Photon., 1, 762-767(2014).
[12] X. Zhang, Y. Xu, W. Yue, Z. Tian, J. Gu, Y. Li, R. Singh, S. Zhang, J. Han, W. Zhang. Anomalous surface wave launching by handedness phase control. Adv. Mater., 27, 7123-7129(2015).
[13] Z. Bomzon, G. Biener, V. Kleiner, E. Hasman. Space-variant Pancharatnam–Berry phase optical elements with computer-generated subwavelength gratings. Opt. Lett., 27, 1141-1143(2002).
[14] G. Biener, A. Niv, V. Kleiner, E. Hasman. Formation of helical beams by use of Pancharatnam–Berry phase optical elements. Opt. Lett., 27, 1875-1877(2002).
[15] L. Marrucci, C. Manzo, D. Paparo. Optical spin-to-orbital angular momentum conversion in inhomogeneous anisotropic media. Phys. Rev. Lett., 96, 163905(2006).
[16] L. Huang, X. Chen, H. Mühlenbernd, G. Li, B. Bai, Q. Tan, G. Jin, T. Zentgraf, S. Zhang. Dispersionless phase discontinuities for controlling light propagation. Nano Lett., 12, 5750-5755(2012).
[17] S. I. Vinitskiĭ, V. L. Derbov, V. M. Dubovik, B. L. Markovski, Y. P. Stepanovskiĭ. Topological phases in quantum mechanics and polarization optics. Sov. Phys. Uspekhi, 33, 403-428(1990).
[18] A. Tomita, R. Y. Chiao. Observation of Berrys topological phase by use of an optical fiber. Phys. Rev. Lett., 57, 937-940(1986).
[19] N. Yu, F. Capasso. Flat optics with designer metasurfaces. Nat. Mater., 13, 139-150(2014).
[20] S. Xiao, J. Wang, F. Liu, S. Zhang, X. Yin, J. Li. Spin-dependent optics with metasurfaces. Nanophotonics, 6, 215-234(2017).
[21] X. Yu, X. Wang, Z. Li, L. Zhao, F. Zhou, J. Qu, J. Song. Spin Hall effect of light based on a surface plasmonic platform. Nanophotonics, 10, 3031-3048(2021).
[22] D. Lin, P. Fan, E. Hasman, M. L. Brongersma. Dielectric gradient metasurface optical elements. Science, 345, 298-302(2014).
[23] G. Li, M. Kang, S. Chen, S. Zhang, E. Y.-B. Pun, K. W. Cheah, J. Li. Spin-enabled plasmonic metasurfaces for manipulating orbital angular momentum of light. Nano Lett., 13, 4148-4151(2013).
[24] G. Zheng, H. Mühlenbernd, M. Kenney, G. Li, T. Zentgraf, S. Zhang. Metasurface holograms reaching 80% efficiency. Nat. Nanotechnol., 10, 308-312(2015).
[25] H. Zhang, M. Kang, X. Zhang, W. Guo, C. Lv, Y. Li, W. Zhang, J. Han. Coherent control of optical spin-to-orbital angular momentum conversion in metasurface. Adv. Mater., 29, 1604252(2017).
[26] P. Genevet, F. Capasso, F. Aieta, M. Khorasaninejad, R. Devlin. Recent advances in planar optics: from plasmonic to dielectric metasurfaces. Optica, 4, 139-152(2017).
[27] Y. Hu, X. Wang, X. Luo, X. Ou, L. Li, Y. Chen, P. Yang, S. Wang, H. Duan. All-dielectric metasurfaces for polarization manipulation: principles and emerging applications. Nanophotonics, 9, 3755-3780(2020).
[28] J. P. Balthasar Mueller, N. A. Rubin, R. C. Devlin, B. Groever, F. Capasso. Metasurface polarization optics: independent phase control of arbitrary orthogonal states of polarization. Phys. Rev. Lett., 118, 113901(2017).
[29] X. Luo, X. Li, M. Pu, Y. Guo, F. Zhang, X. Ma. Symmetric and asymmetric photonic spin-orbit interaction in metasurfaces. Prog. Quantum Electron., 79, 100344(2021).
[30] F. Ding, S. Tang, S. I. Bozhevolnyi. “Recent advances in polarization-encoded optical metasurfaces. Adv. Photon. Res., 2, 2000173(2021).
[31] Y. Qiu, S. Tang, T. Cai, H. Xu, F. Ding. Fundamentals and applications of spin-decoupled Pancharatnam–Berry metasurfaces. Front. Optoelectron., 14, 134-147(2021).
[32] Y. Guo, S. Zhang, M. Pu, Q. He, J. Jin, M. Xu, Y. Zhang, P. Gao, X. Luo. Spin-decoupled metasurface for simultaneous detection of spin and orbital angular momenta via momentum transformation. Light Sci. Appl., 10, 63(2021).
[33] Y. Xu, Q. Li, X. Zhang, M. Wei, Q. Xu, Q. Wang, H. Zhang, W. Zhang, C. Hu, Z. Zhang, C. Zhang, X. Zhang, J. Han, W. Zhang. Spin-decoupled multifunctional metasurface for asymmetric polarization generation. ACS Photon., 6, 2933-2941(2019).
[34] P. Huo, C. Zhang, W. Zhu, M. Liu, S. Zhang, S. Zhang, L. Chen, H. J. Lezec, A. Agrawal, Y. Lu, T. Xu. Photonic spin-multiplexing metasurface for switchable spiral phase contrast imaging. Nano Lett., 20, 2791-2798(2020).
[35] H.-X. Xu, L. Han, Y. Li, Y. Sun, J. Zhao, S. Zhang, C.-W. Qiu. Completely spin-decoupled dual-phase hybrid metasurfaces for arbitrary wavefront control. ACS Photon., 6, 211-220(2018).
[36] T. Li, X. Li, S. Yan, X. Xu, S. Wang, B. Yao, Z. Wang, S. Zhu. Generation and conversion dynamics of dual Bessel beams with a photonic spin-dependent dielectric metasurface. Phys. Rev. Appl., 15, 014059(2021).
[37] C. Zheng, J. Li, G. Wang, J. Li, S. Wang, M. Li, H. Zhao, Z. Yue, Y. Zhang, Y. Zhang, J. Yao. All-dielectric chiral coding metasurface based on spin-decoupling in terahertz band. Nanophotonics, 10, 1347-1355(2021).
[38] H. Zhang, X. Zhang, Q. Xu, C. Tian, Q. Wang, Y. Xu, Y. Li, J. Gu, Z. Tian, C. Ouyang, X. Zhang, C. Hu, J. Han, W. Zhang. High-efficiency dielectric metasurfaces for polarization-dependent terahertz wavefront manipulation. Adv. Opt. Mater., 6, 1700773(2018).
[39] N. Yu, P. Genevet, M. A. Kats, F. Aieta, J.-P. Tetienne, F. Capasso, Z. Gaburro. Light propagation with phase discontinuities: Generalized laws of reflection and refraction. Science, 334, 333-337(2011).
[40] F. Aieta, P. Genevet, M. A. Kat, N. Yu, R. Blanchard, Z. Gaburro, F. Capasso. Aberration-free ultrathin flat lenses and axicons at telecom wavelengths based on plasmonic metasurfaces. Nano Lett., 12, 4932-4936(2012).
[41] H. Zhang, X. Zhang, Q. Xu, Q. Wang, Y. Xu, M. Wei, Y. Li, J. Gu, Z. Tian, C. Ouyang, X. Zhang, C. Hu, J. Han, W. Zhang. Polarization-independent all-silicon dielectric metasurfaces in the terahertz regime. Photon. Res., 6, 24-29(2018).
[42] L. Allen, M. W. Beijersbergen, R. J. C. Spreeuw, J. P. Woerdman. Orbital angular momentum of light and the transformation of Laguerre-Gaussian laser modes. Phys. Rev. A, 45, 8185-8189(1992).
[43] M. Huff. Recent advances in reactive ion etching and applications of high-aspect-ratio microfabrication. Micromachines, 12, 991(2021).
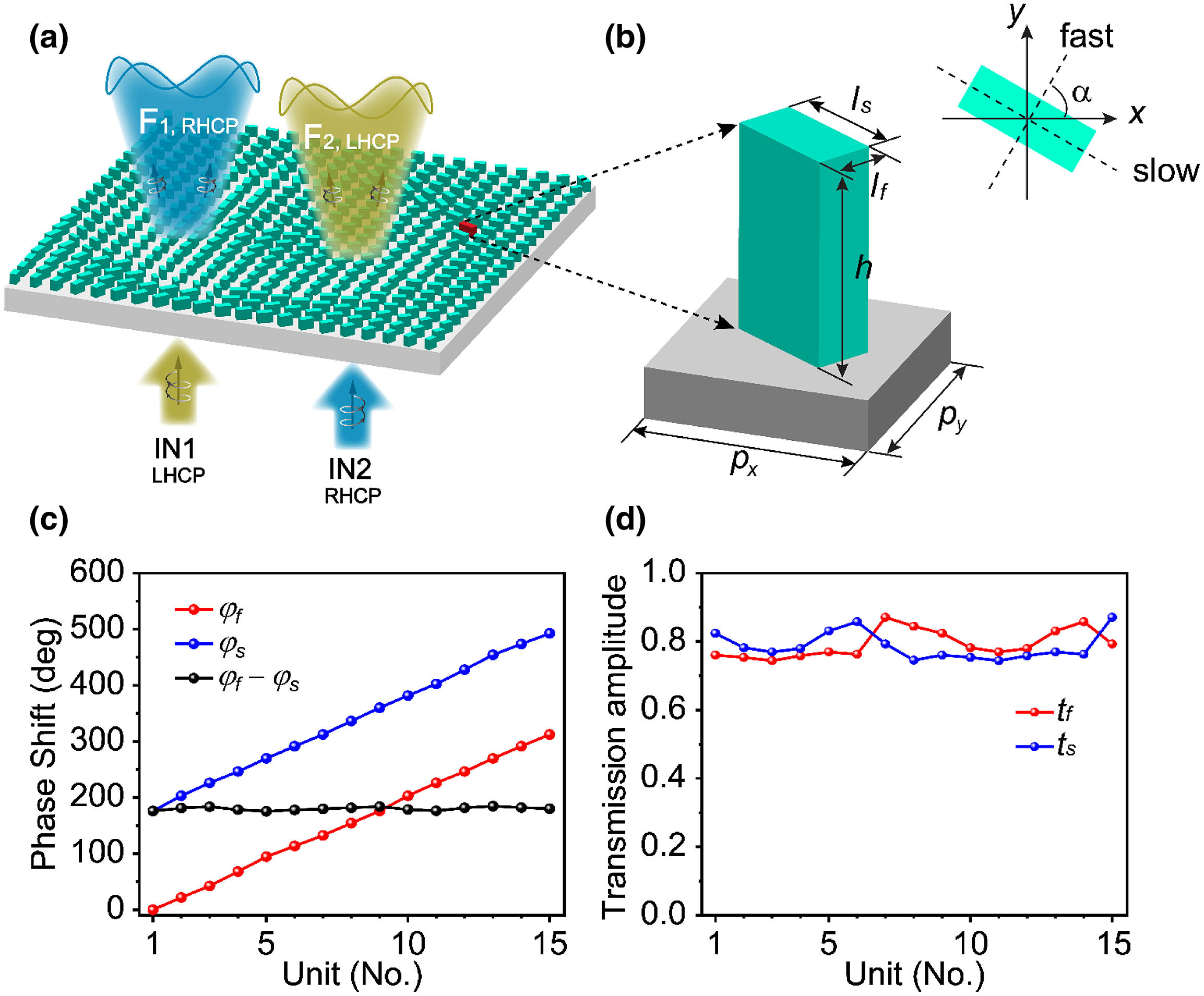
Set citation alerts for the article
Please enter your email address