Xinyu Huang, Xu Han, Yunyun Dai, Xiaolong Xu, Jiahao Yan, Mengting Huang, Pengfei Ding, Decheng Zhang, Hui Chen, Vijay Laxmi, Xu Wu, Liwei Liu, Yeliang Wang, Yang Xu, Yuan Huang. Recent progress on fabrication and flat-band physics in 2D transition metal dichalcogenides moiré superlattices[J]. Journal of Semiconductors, 2023, 44(1): 011901

Search by keywords or author
- Journal of Semiconductors
- Vol. 44, Issue 1, 011901 (2023)
![(Color online) Schematic illustration of the topics of this review, including the fabrication methods and several distinctive properties of the twisted moiré materials[4–6]. ([4] Copyright 2018, American Physical Society. [5] Copyright 2018, American Physical Society. [6] Copyright 2021, Nature Publishing Group (NPG).)](/richHtml/jos/2023/44/1/011901/jos_44_1_011901_f1.jpg)
Fig. 1. (Color online) Schematic illustration of the topics of this review, including the fabrication methods and several distinctive properties of the twisted moiré materials[4–6]. ([4] Copyright 2018, American Physical Society. [5] Copyright 2018, American Physical Society. [6] Copyright 2021, Nature Publishing Group (NPG).)
![(Color online) Mechanism of Au-film-assisted exfoliation technology and some examples of exfoliated 2D crystals. (a) Part of the periodic table, showing the elements involved in most 2D materials between groups 4 (IVB) and 17 (VIIA). Most of the layered crystals are composed of the elements with pink and green colors, which have strong interaction with Au. (b) Schematic of the interaction mechanism between layered crystal and Au. Once the interface interaction energy is larger than the interlayer interaction, monolayer flakes can be exfoliated. (c) Schematic illustration of the Au-film-assisted exfoliation process. (d) Optical images of large exfoliated 2D flakes[50]. Copyright 2022, Nature Publishing Group (NPG).](/richHtml/jos/2023/44/1/011901/jos_44_1_011901_f2.jpg)
Fig. 2. (Color online) Mechanism of Au-film-assisted exfoliation technology and some examples of exfoliated 2D crystals. (a) Part of the periodic table, showing the elements involved in most 2D materials between groups 4 (IVB) and 17 (VIIA). Most of the layered crystals are composed of the elements with pink and green colors, which have strong interaction with Au. (b) Schematic of the interaction mechanism between layered crystal and Au. Once the interface interaction energy is larger than the interlayer interaction, monolayer flakes can be exfoliated. (c) Schematic illustration of the Au-film-assisted exfoliation process. (d) Optical images of large exfoliated 2D flakes[50]. Copyright 2022, Nature Publishing Group (NPG).
![(Color online) Ag-assisted exfoliation procedures and optical images of exfoliated samples. (a) Schematic illustration of the exfoliation procedures. (b) Exfoliated macroscopic MoS2 and WS2 on 15 nm Ag film supported by SiO2/Si substrates, and bulk crystals on PDMS tapes. (c) Exfoliated macroscopic MoS2 supported by sapphire substrates. (d) Exfoliated MoS2 supported by elastic PET substrates. (e) Exfoliated MoS2 on Ag/epoxy/glass slide substrate. (f) Optical microscope images of some 2D crystals exfoliated on 15 nm Ag film, including MoS2, WS2, 1T-WTe2, and BP. (g) Optical microscope images of exfoliated millimeter size 2D crystals on 5 nm Ag film, including ReSe2, Fe3GeTe2, FeSe, and TaS2. (h, i) Optical microscope images of exfoliated millimeter size MoS2 on sapphire substrate and TS Ag, respectively. (j) Optical microscope and PL mapping images of exfoliated monolayer WS2 on 15 nm Ag film with hole array, the scale bars in the two images are 40 and 20μm, respectively[51]. Copyright 2022, Wiley Online Library.](/Images/icon/loading.gif)
Fig. 3. (Color online) Ag-assisted exfoliation procedures and optical images of exfoliated samples. (a) Schematic illustration of the exfoliation procedures. (b) Exfoliated macroscopic MoS2 and WS2 on 15 nm Ag film supported by SiO2/Si substrates, and bulk crystals on PDMS tapes. (c) Exfoliated macroscopic MoS2 supported by sapphire substrates. (d) Exfoliated MoS2 supported by elastic PET substrates. (e) Exfoliated MoS2 on Ag/epoxy/glass slide substrate. (f) Optical microscope images of some 2D crystals exfoliated on 15 nm Ag film, including MoS2, WS2, 1T-WTe2, and BP. (g) Optical microscope images of exfoliated millimeter size 2D crystals on 5 nm Ag film, including ReSe2, Fe3GeTe2, FeSe, and TaS2. (h, i) Optical microscope images of exfoliated millimeter size MoS2 on sapphire substrate and TS Ag, respectively. (j) Optical microscope and PL mapping images of exfoliated monolayer WS2 on 15 nm Ag film with hole array, the scale bars in the two images are 40 and 20μm, respectively[51]. Copyright 2022, Wiley Online Library.
![(Color online) Fabrication process and characterization of suspended 2D materials. (a) Schematic images for preparing suspended samples. (b–d) Optical images of exfoliated graphene, MoS2 and WSe2 on different patterned substrates, including rectangle, Hall bar and circular hole structures. (e) PL mapping image of suspended monolayer WSe2[48]. Copyright 2022, Wiley Online Library.](/Images/icon/loading.gif)
Fig. 4. (Color online) Fabrication process and characterization of suspended 2D materials. (a) Schematic images for preparing suspended samples. (b–d) Optical images of exfoliated graphene, MoS2 and WSe2 on different patterned substrates, including rectangle, Hall bar and circular hole structures. (e) PL mapping image of suspended monolayer WSe2[48]. Copyright 2022, Wiley Online Library.
![(Color online) Tear-rotate-stack method for fabricating 2D twisted heterostructure. (a) Schematic of cutting the 2D materials with femtosecond laser to get the straight edge for twist angle reference[54]. Copyright 2016, Wiley Online Library. (b) Preparing the twisted bilayer graphene with desired twist angle using the tear-rotate-stack method[53]. Copyright 2017, National Academy of Sciences (NAS). (c) The tear-rotate-stack method to fabricate the twisted MoS2 homostructures from the as-grown wafer MoS2 monolayer. (d) Optical image of 30° twisted bilayer MoS2[55]. Copyright 2020, Nature Publishing Group (NPG).](/Images/icon/loading.gif)
Fig. 5. (Color online) Tear-rotate-stack method for fabricating 2D twisted heterostructure. (a) Schematic of cutting the 2D materials with femtosecond laser to get the straight edge for twist angle reference[54]. Copyright 2016, Wiley Online Library. (b) Preparing the twisted bilayer graphene with desired twist angle using the tear-rotate-stack method[53]. Copyright 2017, National Academy of Sciences (NAS). (c) The tear-rotate-stack method to fabricate the twisted MoS2 homostructures from the as-grown wafer MoS2 monolayer. (d) Optical image of 30° twisted bilayer MoS2[55]. Copyright 2020, Nature Publishing Group (NPG).
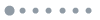
Fig. 6. (Color online) Schematic illustration of the band structure and Hubbard model simulation of the TMD moiré systems. (a) showing the narrowing of bandwidth with a larger wavelength (λ) of the periodic potential well for 1D lattice in the nearly-free electron approximation. The additional periodic potential folds the bands into a mini-Brillouin zone with boundary at ±π/λ. The lowest band (orange) becomes narrower with the bandwidth tuned by λ. (b) Typical band alignment of angle-aligned heterobilayers and AB stacked homobilayers with SU(2) and SU(4) symmetry, respectively. (c) Schematic illustration of the inter-site hopping termt, on-site Coulomb repulsionU, and inter-site Coulomb repulsionV. (d) Quantum phase diagram of the half-filled triangular lattice.
![(Color online) Simulation of Hubbard model in the strong correlation limit. (a) Rydberg sensing of the abundant correlated insulating states in WSe2/WS2 described by a single-band extended Hubbard model with SU(2) symmetry. (b) Charge configuration at several typical fillings in (a)[78]. (c) Electric-field-controlled layer polarization of t-WSe2 (mapped to a SU(4) bilayer Hubbard model) probed by the moiré exciton resonance. The dashed line denotes the boundary of fully polarized states (|P| = 1, in blue). (d) Doping dependence of MCD (proportional to sample magnetization) withP = 0 (blue) andP = 1 (black)[84]. The vanishing magnetization atν = 2,P = 1 is related to the formation of antiferromagnetic order. (e) Charge/spin configuration of an excitonic insulator and an antiferromagnetic insulator in AB-stacked t-WSe2.](/Images/icon/loading.gif)
Fig. 7. (Color online) Simulation of Hubbard model in the strong correlation limit. (a) Rydberg sensing of the abundant correlated insulating states in WSe2/WS2 described by a single-band extended Hubbard model with SU(2) symmetry. (b) Charge configuration at several typical fillings in (a)[78]. (c) Electric-field-controlled layer polarization of t-WSe2 (mapped to a SU(4) bilayer Hubbard model) probed by the moiré exciton resonance. The dashed line denotes the boundary of fully polarized states (|P| = 1, in blue). (d) Doping dependence of MCD (proportional to sample magnetization) withP = 0 (blue) andP = 1 (black)[84]. The vanishing magnetization atν = 2,P = 1 is related to the formation of antiferromagnetic order. (e) Charge/spin configuration of an excitonic insulator and an antiferromagnetic insulator in AB-stacked t-WSe2.
![(Color online) Hubbard model physics with an intermediate correlation[87]. (a) Longitudinal resistanceρxx atT = 20 K,B = 0 T in angle-aligned 3L-MoTe2/WSe2. A resistance peak is discovered atν = 1. (b) Evolution of the gates-dependentρxx as a function of temperature. (c) Temperature dependence ofρxx atν = 1 andD = 1.313 V/nm. At low temperatures, theρxx accords well with the blue dashed curve (∝T2) denoting a Fermi-liquid behavior. (d) Magnetic-field dependence of theν = 1 resistance atT = 0.3 K. Sharp resistance peaks occur above the critical magnetic fieldBc ≈ 6 T. (e) Phase diagram of 3L-MoTe2/WSe2 at half filling.](/Images/icon/loading.gif)
Fig. 8. (Color online) Hubbard model physics with an intermediate correlation[87]. (a) Longitudinal resistanceρxx atT = 20 K,B = 0 T in angle-aligned 3L-MoTe2/WSe2. A resistance peak is discovered atν = 1. (b) Evolution of the gates-dependentρxx as a function of temperature. (c) Temperature dependence ofρxx atν = 1 andD = 1.313 V/nm. At low temperatures, theρxx accords well with the blue dashed curve (∝T2) denoting a Fermi-liquid behavior. (d) Magnetic-field dependence of theν = 1 resistance atT = 0.3 K. Sharp resistance peaks occur above the critical magnetic fieldBc ≈ 6 T. (e) Phase diagram of 3L-MoTe2/WSe2 at half filling.
![(Color online) Kane–Mele–Hubbard model in AB stacked WSe2/MoTe2[6]. (a) Schematic illustration of the electric-field-induced topological phase transitions. (b) Longitudinal resistanceRxx atT = 300 mK,B = 0 T, with the green dashed circle denoting the quantum anomalous Hall region. (c) Hall (Rxy) and (d) longitudinal (Rxx) resistances versusB-field in the QAH region. QuantizedRxy and vanishingRxx are observed at low temperatures. (e) Electric-field dependence ofRxx atν = 1 under zero magnetic field at varying temperatures. (f) Electric-field dependence of the extracted charge gap (∆C by thermal activation fits to the resistance data, ∆tr by direct compressibility measurements) atν = 1 from the Mott insulating region to the QAH region and the metallic region.](/Images/icon/loading.gif)
Fig. 9. (Color online) Kane–Mele–Hubbard model in AB stacked WSe2/MoTe2[6]. (a) Schematic illustration of the electric-field-induced topological phase transitions. (b) Longitudinal resistanceRxx atT = 300 mK,B = 0 T, with the green dashed circle denoting the quantum anomalous Hall region. (c) Hall (Rxy) and (d) longitudinal (Rxx) resistances versusB-field in the QAH region. QuantizedRxy and vanishingRxx are observed at low temperatures. (e) Electric-field dependence ofRxx atν = 1 under zero magnetic field at varying temperatures. (f) Electric-field dependence of the extracted charge gap (∆C by thermal activation fits to the resistance data, ∆tr by direct compressibility measurements) atν = 1 from the Mott insulating region to the QAH region and the metallic region.
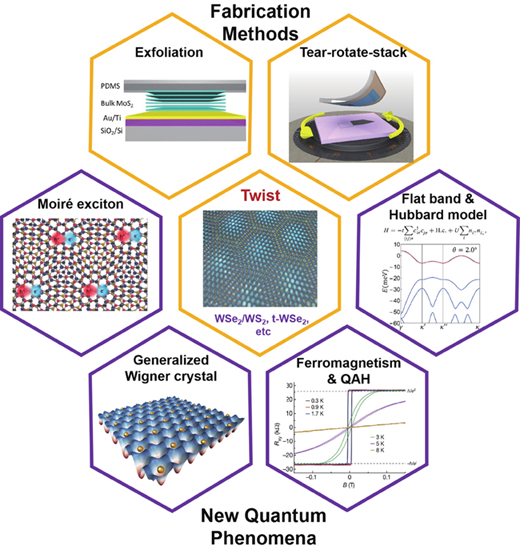
Set citation alerts for the article
Please enter your email address