
- Photonics Research
- Vol. 10, Issue 6, 1325 (2022)
Abstract
1. INTRODUCTION
Distributed optical fiber sensing has been investigated extensively for various engineering applications due to its unique advantages, such as immunity to electromagnetic interference, small size, and the capability of long-term monitoring [1–3]. Traditional distributed optical fiber sensors are commonly realized by exploring Raman scattering [3], Rayleigh backscattering [4], or backward stimulated Brillouin scattering (BSBS) [5] in optical fibers. In such distributed sensors, light does not interact with the environment directly, and sensing is achieved because the environment affects the fiber property, which modifies the characteristics of the scattering effects. Consequently, the physical quantities that can be measured are limited to temperature, strain, and vibration, and the parameters derived from them. Direct interaction between light and the environment can be realized using fiber tapers or D-shaped fibers. By facilitating refractive index, chemical, and biomedical sensing, these sensors can be applied in the petrochemical industry or to guarantee food safety [6–8]. However, the compromised mechanical strength, large insertion loss, and complicated structure make large-scale multiplexing of the sensors difficult.
In recent years, a novel optical fiber sensing method has been proposed by exploring forward stimulated Brillouin scattering (FSBS) [9]. In this nonlinear scattering process, radial and torsional-radial acoustic modes are induced optically. These acoustic modes lead to refractive index modulation of the optical fiber due to photo-elasticity, resulting in phase and/or polarization modulation on the forward-propagating light. As radial and torsional-radial modes are sensitive to the acoustic property of the surrounding liquid, the acoustic impedance of the liquid can be obtained from the FSBS spectrum of a specific acoustic mode by demodulating the induced phase or polarization modulation [10,11].
Apart from no longer requiring complicated sensor structure design, FSBS-based acoustic impedance sensing is promising for the realization of sensor multiplexing or even distributed sensing. Several techniques have been developed based on different FSBS excitation and detection schemes. With continuous-wave (CW) light excitation, a multi-point sensor is realized with a frequency-division multiplexing (FDM) scheme based on spontaneous forward Brillouin scattering, reporting spatial resolution of 0.8 m with limited multiplexing capacity [12]. By injecting a set of dual-frequency pulses with a frequency difference matching a specific acoustic mode, continuous energy transfer between the two pulses can be facilitated through FSBS. Distributed FSBS measurement can thus be realized by exploring opto-mechanical time-domain reflectometry (OM-TDR) [13,14] or OM time-domain analysis (OM-TDA) [15–17], achieving spatial resolution of up to 0.8 m. However, due to the small frequency interval between the two pulses, for each data point of the FSBS spectrum, the BSBS spectrum has to be constructed first to measure each component separately in virtue of its narrowband filtering and amplification capability. Consequently, two round frequency sweeps are required for the reconstruction of FSBS and BSBS spectra, making sensing signal acquisition and processing very time consuming. Using an excitation pulse with amplitude modulation at a specific frequency, the intensity of FSBS can be obtained via the evolution of refractive index modulation depth or probe pulse central frequency shift along the fiber [18,19], achieving a spatial resolution up to 0.8 m based on Brillouin optical-fiber TDA (BOTDA). However, the reconstruction of BSBS and FSBS spectra is also necessary. The sensing system based on Brillouin optical TDR (BOTDR) has achieved 8-m spatial resolution [19] with only one round of frequency sweep to reconstruct the FSBS spectrum. Nonetheless, excessive reliance on a bandpass filter to obtain each component’s intensity limits its practical application.
Sign up for Photonics Research TOC. Get the latest issue of Photonics Research delivered right to you!Sign up now
By coherently detecting the Rayleigh backscattering signal generated from an optical pulse under FSBS influence, it seems possible to obtain the refractive index modulation depth. In that case, the additional frequency sweep required for BSBS-based detection becomes unnecessary. However, due to the weak and random nature of Rayleigh backscattering in standard single-mode fibers (SMFs), the sensing signal level is too low to achieve the signal-to-noise ratio (SNR) required for acoustic impedance measurement, even with coherent detection.
In this work, we propose and demonstrate a distributed acoustic impedance sensing technique based on coherent detection, by generating an enhanced backward-propagating sensing signal using an identical chirped fiber Bragg grating (CFBG) array. The excitation method proposed by Chow
2. PRINCIPLE
In this work, FSBS is invoked using an optical pulse with single-frequency amplitude modulation near the resonance frequency of a radial mode. For clarity, the radial mode is denoted by
In Eq. (3),
Equation (4) implies that the sideband intensities, determined by
3. EXPERIMENTAL SETUP AND RESULTS
The experimental setup depicted by Fig. 1 is adopted to demonstrate the coherent-detection-based distributed acoustic impedance sensing technique. To generate the excitation pulse, the output of laser#1 is first modulated using a semiconductor optical amplifier (SOA#1) in a rectangular pulse with 250-ns pulse width. An electro-optic modulator (EOM) is then applied to incorporate single-frequency amplitude modulation at
Figure 1.Experimental setup of the coherent-detection-based distributed acoustic impedance sensing system enabled by a grating array. SOA, semiconductor optical amplifier; EOM, electro-optic modulator; EDFA, erbium-doped optical fiber amplifier; FC, fiber coupler; CFBGs, chirped fiber Bragg gratings; FUT, fiber under test; ISO, isolator; AOM, acousto-optic modulator; PC, polarization controller; PD, photodetector; DAQ, data acquisition card.
Figure 2.Partial temporal waveform of the excitation and probe pulses, presenting 10-ns guard interval.
To recover the distributed refractive index modulation depth, the amplitudes of at least three spectral peaks corresponding to
Beat Frequencies Corresponding to
Sideband Order | Beat Frequency (MHz) | ||||
---|---|---|---|---|---|
0 | 200.00 | 200.00 | 200.00 | 200.00 | 200.00 |
1 | 218.02 | 265.88 | 313.72 | 361.56 | 409.40 |
618.02 | 665.88 | 713.72 | 761.56 | 809.40 | |
2 | 636.04 | 731.76 | 827.44 | 923.12 |
The resonance frequencies of the
With
Figure 3.(a) Beat signal segment and (b) normalized spectrum corresponding to a sensing fiber section between two CFBGs, when the excitation pulse is turned off.
Figure 4.(a) Beat signal segment and (b) normalized spectrum corresponding to a sensing fiber section between two CFBGs, when the excitation pulse is turned on.
For comparison, a 600-m standard SMF is used to obtain the Rayleigh backscattering of the probe signal. To create a region with different environmental acoustic impedance, the coating of the fiber section between 317 m and 341 m is stripped. The sensing system is the same as that depicted by Fig. 1, except that the settings of the EDFAs are reconfigured to provide higher output power, so that the Rayleigh backscattering signal is strong enough for the detection. The average powers of the excitation and probe pulses are set at 10 dBm and
Figure 5.(a) Beat signal of Rayleigh scattering as the excitation pulse is turned on and (b) frequency-domain diagram. (c) Part of the beat signal and (d) its corresponding frequency-domain diagram.
Figure 5(b) shows the FFT result of the time-domain signal corresponding to the entire sensing fiber. The beat frequency components at 351.56 MHz, 761.56 MHz, and 923.12 MHz can also be identified, but with severely degraded quality. Furthermore, if we look at the FFT of a signal segment corresponding to a 5-m fiber section, as shown in Figs. 5(c) and 5(d), even the frequency components corresponding to
Figure 6 shows the waveform of the beat signal generated by the sensing signal from the entire CFBG array. To facilitate the subsequent signal processing, the beat signal is divided into 100 segments, each containing a single beat pulse from a CFBG, as indicated by the different colors. It is observed that the amplitudes of the beat pulses are not uniform, which is attributed to the polarization fading effect along the fiber. Since the calculation of additional phase factor
Figure 6.Beat pulses generated by the entire sensing fiber.
To validate the capability of the CFBG-array-enabled distributed acoustic impedance sensing, the coating of the fiber section between 310 m and 315 m is stripped. As the acoustic impedance mismatch between silica and air is much larger than that between silica and the polymer coating, the induced acoustic mode would be stronger, leading to more intense phase modulation at this fiber section, which should be revealed in the evolution of additional phase factor
Figure 7.Spectra of beat pulses from the entire sensing fiber.
Figure 8.Evolution of amplitudes of frequency components at
Figure 9.(a) Averaged additional phase factor
Distributed measurement of the environmental acoustic impedance is then demonstrated by sweeping the modulation frequency
Figure 10.Distributed FSBS spectra when the stripped fiber section is placed in (a) air, (b) anhydrous ethanol, and (c) water.
Figure 11.FSBS spectra of the stripped fiber section when it is placed in (a) air, (b) anhydrous ethanol, and (c) water.
Immersing the stripped fiber section in anhydrous ethanol, the distributed FSBS spectra are reconstructed and plotted in Fig. 10(b), and the FSBS spectrum at the corresponding location is plotted in Fig. 11(b). By fitting to a Lorentzian shape, the FWHM spectral width is found to be
4. CONCLUSION AND DISCUSSION
In this work, a distributed acoustic impedance sensing system is demonstrated based on FSBS and coherent detection. By adopting a CFBG array, a backward-propagating sensing signal with notably improved SNR is generated, enabling the measurement of phase modulation depth and thus the refractive index modulation depth along the sensing fiber, which is induced by a specifically excited
Since the time-domain sensing signals are transformed into frequency-domain signals for processing, the spectral resolution needs to be small enough so that the different spectral peaks can be differentiated. Therefore, the pulse width of the probe cannot be too short, which limits the spatial resolution of the system. In other words, even though in practice, the grating spacing corresponds to the spatial resolution of the sensing system, it is not what restricts the spatial resolution. To improve spatial resolution, wavelength-division multiplexed CFBGs can be incorporated into the time-division multiplexed array [24], facilitating a hybridly multiplexed sensing system.
In a CFBG array, the signal crosstalk generated by multiple reflections scales up as the number of gratings increases. Such crosstalk will eventually affect the SNR of the sensing signal, especially that at the end of the fiber. However, due to the low reflectivity of the CFBGs (
Currently, the inscription of FBGs in SMFs has been demonstrated using the phase-mask method [26] and point-by-point fabrication using femtosecond lasers [27]. However, this specialty fiber is still almost commercially unavailable, which could be a demerit of the proposed method.
References
[1] X. Bao, L. Chen. Recent progress in distributed fiber optic sensors. Sensors, 12, 8601-8639(2012).
[2] A. H. Hartog. An Introduction to Distributed Optical Fibre Sensors(2017).
[3] P. Lu, N. Lalam, M. Badar, B. Liu, B. T. Chorpening, M. P. Buric, P. R. Ohodnicki. Distributed optical fiber sensing: review and perspective. Appl. Phys. Rev., 6, 041302(2019).
[4] X. Bao, Y. Wang. Recent advancements in Rayleigh scattering-based distributed fiber sensors. Adv. Devices Instrum., 2021, 8696571(2021).
[5] X. Bao, Z. Zhou, Y. Wang. Review: distributed time-domain sensors based on Brillouin scattering and FWM enhanced SBS for temperature, strain and acoustic wave detection. PhotoniX, 2, 14(2021).
[6] Z. Ding, K. Sun, K. Liu, J. Jiang, D. Yang, Z. Yu, J. Li, T. Liu. Distributed refractive index sensing based on tapered fibers in optical frequency domain reflectometry. Opt. Express, 26, 13042-13054(2018).
[7] P. Xu, X. Yu, Z. Chen, L. Sheng, J. Liu, S. Zhou, K. Wen, O. Xu, X. Dong, J. Yang, Y. Qin. Distributed refractive index sensing based on bending-induced multimodal interference and Rayleigh backscattering spectrum. Opt. Express, 29, 21530-21538(2021).
[8] C. Gong, Y. Gong, X. Zhao, Y. Luo, Q. Chen, X. Tan, Y. Wu, X. Fan, G. D. Peng, Y. J. Rao. Distributed fibre optofluidic laser for chip-scale arrayed biochemical sensing. Lab Chip, 18, 2741-2748(2018).
[9] Y. Antman, A. Clain, Y. London, A. Zadok. Optomechanical sensing of liquids outside standard fibers using forward stimulated Brillouin scattering. Optica, 3, 510-516(2016).
[10] N. Hayashi, Y. Mizuno, K. Nakamura, S. Y. Set, S. Yamashita. Experimental study on depolarized GAWBS spectrum for optomechanical sensing of liquids outside standard fibers. Opt. Express, 25, 2239-2244(2017).
[11] D. M. Chow, L. Thévenaz. Forward Brillouin scattering acoustic impedance sensor using thin polyimide-coated fiber. Opt. Lett., 43, 5467-5470(2018).
[12] Z. Zheng, Z. Li, X. Fu, L. Wang, H. Wang. Multipoint acoustic impedance sensing based on frequency-division multiplexed forward stimulated Brillouin scattering. Opt. Lett., 45, 4523-4526(2020).
[13] G. Bashan, H. H. Diamandi, Y. London, E. Preter, A. Zadok. Optomechanical time-domain reflectometry. Nat. Commun., 9, 2991(2018).
[14] H. H. Diamandi, Y. London, G. Bashan, A. Zadok. Distributed opto-mechanical analysis of liquids outside standard fibers coated with polyimide. APL Photon., 4, 016105(2019).
[15] C. Pang, Z. Hua, D. Zhou, H. Zhang, L. Chen, X. Bao, Y. Dong. Opto-mechanical time-domain analysis based on coherent forward stimulated Brillouin scattering probing. Optica, 7, 176-184(2020).
[16] Z. Hua, D. Ba, D. Zhou, Y. Li, Y. Wang, X. Bao, Y. Dong. Non-destructive and distributed measurement of optical fiber diameter with nanometer resolution based on coherent forward stimulated Brillouin scattering. Light Adv. Manuf., 2, 25(2021).
[17] Y. K. Dong, Z. Hua, D. Ba, Y. Li. Polarization separation assisted opto-mechanical time-domain analysis with sub-meter resolution. Opt. Lett., 46, 5886-5889(2021).
[18] D. M. Chow, Z. Yang, M. A. Soto, L. Thevenaz. Distributed forward Brillouin sensor based on local light phase recovery. Nat. Commun., 9, 2990(2018).
[19] S. Zaslawski, Z. Yang, S. Wang, L. Thévenaz. Distributed forward stimulated Brillouin scattering measurement using broadband BOTDR. Proc. SPIE, 11199, 1119923(2019).
[20] X. Fu, J. Wu, Z. Li, Y. Tong, X. Gui, H. Wang. Fiber-based large dynamic range vibration sensing with dual-wavelength phase unwrapping. J. Lightwave Technol., 37, 6090-6096(2019).
[21] J. Wang, Z. Li, X. Fu, X. Gui, J. Zhan, H. Wang, D. Jiang. High-sensing-resolution distributed hot spot detection system implemented by a relaxed pulsewidth. Opt. Express, 28, 16045-16056(2020).
[22] R. M. Shelby, M. D. Levenson, P. W. Bayer. Guided acoustic-wave Brillouin scattering. Phys. Rev. B, 31, 5244-5252(1985).
[23] M. Alem, M. A. Soto, M. Tur, L. Thévenaz. Analytical expression and experimental validation of the Brillouin gain spectral broadening at any sensing spatial resolution. 25th Optical Fiber Sensors Conference (OFS), 1-4(2017).
[24] Y. Ou, C. Zhou, L. Qian, D. Fan, C. Cheng, H. Guo, Z. Xiong. Large WDM FBG sensor network based on frequency-shifted interferometry. IEEE Photon. Technol. Lett., 29, 535-538(2017).
[25] J. Wu, Z. Li, X. Fu, M. Fan, X. Gui, H. Wang. High dynamic range distributed acoustic sensing based on dual-wavelength fiber Bragg grating pairs. Opt. Lett., 46, 4402-4405(2021).
[26] H. Guo, J. Tang, X. Li, Y. Zheng, H. Yu, H. Yu. On-line writing identical and weak fiber Bragg grating arrays. Chin. Opt. Lett., 11, 030602(2013).
[27] B. Xu, J. He, B. Du, X. Xiao, X. Xu, C. Fu, J. He, C. Liao, Y. Wang. Femtosecond laser point-by-point inscription of an ultra-weak fiber Bragg grating array for distributed high-temperature sensing. Opt. Express, 29, 32615-32626(2021).
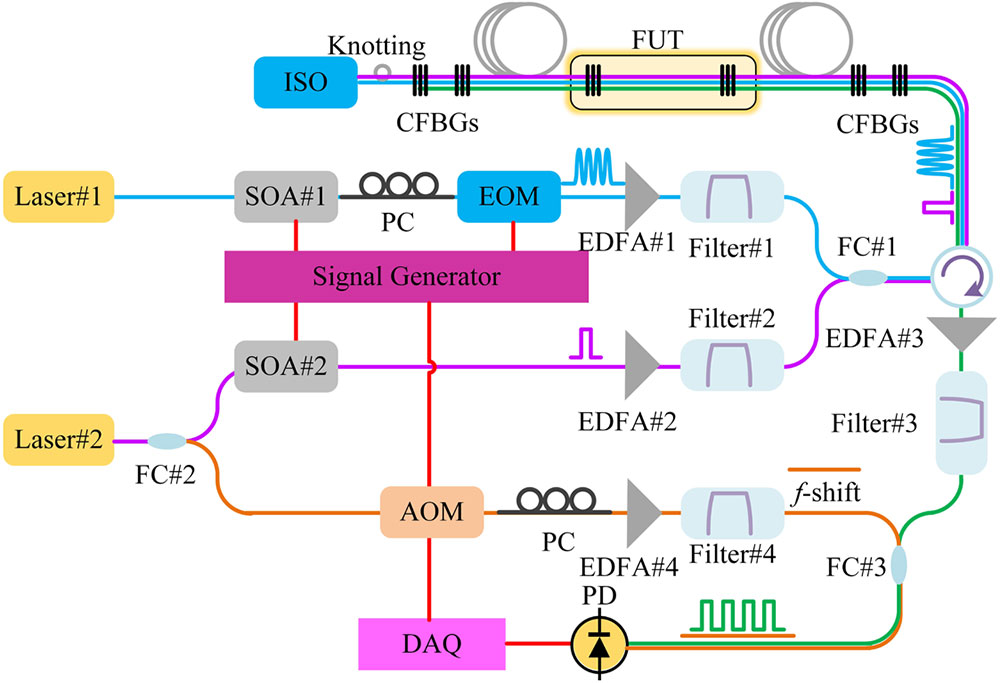
Set citation alerts for the article
Please enter your email address