
- Photonics Research
- Vol. 10, Issue 1, 230 (2022)
Abstract
1. INTRODUCTION
Nonlinear optics plays an important role in modern science and technology [1–3]. Four-wave mixing (FWM) is an important third-order nonlinear optical process, owning wide applications in many areas. Besides its significance in generating quantum-correlated beams and phase-conjugated beams [4,5], FWM also serves as an effective spectroscopic method of many advantages. Resulting from a fully resonant process, FWM spectroscopy has high signal-to-noise ratios (SNRs), which allows for the sensitive and selective detection of stable and transient molecules [6]. Furthermore, the FWM signal is coherent and therefore the entire signal can be collected (rather than a small fraction as compared to an incoherent process like Raman scattering or laser-induced fluorescence) [7]. In addition, the FWM signal is separated from the input beams in direction and thus free from parasitic background fluorescence under proper phase-matching conditions, which further enhances the SNR and makes remote probing possible [8]. So far, FWM spectroscopy has been successfully applied in transient species analysis during combustion [9], isotope ratio measurement [10], and investigation of the energetic structure or dynamic processes of molecules [11,12].
As FWM in many systems is polarization sensitive, polarization-resolved FWM spectral technology is of particular importance. For example, it can be used to reveal the structure and molecular orientation in complex systems [13,14], such as proteins, lipids, and cell membranes. Munhoz and Rigneault have shown that polarization-resolved FWM is a powerful technique for retrieving the even orders of symmetry up to the fourth order in thick collagenous tissues [15]. Polarization-resolved FWM can also be used to determine the third-order nonlinear optical tensor of various media, including transient systems, such as ionized atmospheric air [16] and plasmon excitation on flat graphene [17]. In addition, polarization-resolved FWM is a significant tool for studying the complicated dynamics of nonlinear optical processes, e.g, interwell carrier dynamics [18], ultrafast dynamics in single gold nanoparticles [19], excitonic dephasing [20].
The polarization-resolved FWM is commonly realized by scanning the polarization of incident beams and detecting the signals in sequence. However, such shot-to-shot methods might cause irreversible damage to the samples, and therefore it is not suitable for those samples with poor light stability [15]. Moreover, the modulation speed of the commonly used polarization modulator, such as the electro-optic modulator (EOM), is less than 100 MHz, and thus the transient processes occurring in 10 ns cannot be resolved by shot-to-shot polarization-resolved FWM. Previously, Shalit and Prior proposed an idea of single-scan polarization-resolved FWM where a single incident pulse is spatially encoded with various states of polarization for FWM generation [21]. They also designed an experimental scheme in which an echelon mirror is used to split a pulse into multiple sub-pulses, and then a set of spatial light modulators are employed to modulate the sub-pulses into various states of polarization. However, no results have been reported based on such an experimental geometry.
Sign up for Photonics Research TOC. Get the latest issue of Photonics Research delivered right to you!Sign up now
The vector fields are inherently characterized by space-variant states of polarization. In recent years, vector fields have attracted great attention and have had wide applications in optical micro-manipulation and trapping [22], high-resolution imaging [23], quantum communication [24], etc. Nonuniform spatial polarization provides researchers with one more degree of freedom in studying and utilizing the light–matter interaction. For example, based on the nonlinear interaction of the vector beam and the media, people are able to realize spatial filtering of the vector probe beam based on saturated absorption [25], generate specially correlated radially polarized beams [26], generate the dressed vortex FWM signal and study the modulation effect of the vortex beam on the signal [27], manipulate and select the spatial polarization distribution of a beam [28], etc. We previously realized multi-wave mixing (MWM) generation using a single vector beam, in which we split a vector beam into multiple parts by a polarizer and then focused the multiple parts into the sample to realize MWM [29].
In this work, we propose a new scheme of the single-scan polarization-resolved degenerate four-wave mixing (DFWM) based on a vector field and demonstrate its feasibility with the atomic Rb vapor sample. In the experimental configuration, two pump beams are kept linearly polarized and a vector beam is employed as the probe beam. Utilizing the space-variant polarization of the probe beam, the measurement of the single-scan polarization-resolved spectrum is easily achieved, since the complicated polarization encoded process is avoided. This work not only provides a simple but efficient single-scan polarization-resolved DFWM method, but also provides a method for designing other single-scan polarization-resolved spectral or imaging methods, which would be of significance for low light stability and fast optical processes.
2. EXPERIMENTAL SETUP
Figure 1(a) shows the scheme of our experimental setup. The output from a wavelength tunable continuous-wave (CW) Ti: sapphire laser (Spectra-Physics, Matisse TR) with a spectral linewidth of
Figure 1.(a) Scheme of the experimental setup. Ti:S, Ti:sapphire laser; VR, vortex retarder; HR, high reflection mirror; PBS, polarization beam splitter; and HWP, half-wave plate. (b) Phase-matching configuration of forward four-wave mixing geometry. (c) The related energy level structures of
The beams
The Rb sample cell (15 mm in length and 20 mm in diameter) contains the Rb substrate and can be heated by a heater belt to produce Rb atomic vapor. During the experiments, the temperature of the cell was kept at 338.15 K, and the Rb atomic number density is approximated to be
As for the detecting part, the images of the DFWM signal are captured by a CMOS camera. A spatial filter is placed in front of the CMOS camera to filter out the incident beams. A polarization analyzer is inserted before the CMOS camera when we determine the polarization distribution of the signal beam (not shown). The polarization analyzer we used is a Glan prism.
3. THEORETICAL CONSIDERATIONS
The third-order nonlinear polarization is deduced with density-matrix formalism
Then, the expression of the third-order density matrix elements [32] can be derived using these chains
For DFWM, the frequencies of three incident beams are the same:
The coupling wave equation under the slowly varying amplitude approximation is
To clearly describe the interaction between the atom and the polarized beams, we discuss the situations when the polarization state of
As shown in Fig. 1(c), three transitions
Figure 2.Possible transition paths at different polarization configurations from
It is seen from Fig. 2 that the transition paths are different for different polarization states of
4. RESULTS AND DISCUSSION
To determine the dependence of the DFWM signal on the polarization state of the probe beam
Figure 3.Normalized DFWM signal intensity with respect to the rotation angle of the HWP varying the polarization of
Theoretically, when a linearly polarized beam passes through an HWP, its two projected components are
From the above study, we know that the DFWM signal of the Rb atoms is quite sensitive to the polarization of the probe beam
The polarization distribution of the vector optical field can be varied by rotating the fast axis of the VR. The typical radial and angular vector fields generated are shown in Fig. 4(a) I and IV, respectively, in which the arrows stand for the polarization distribution of the fields, which can be determined through an analyzer. Figure 4(a) II, III, V, and VI show the beam images after the analyzer. Then, keeping the pump beams
Figure 4.Polarization distribution of the single-scan DFWM signal. (a) Beam images of the vector optical field and its corresponding images after the analyzer. (b) Single-scan DFWM signal image when
To find the relationship between the space-variant intensity of the DFWM signal and the space-variant polarization of
Figure 5.DFWM signal images after a polarization analyzer: (a)
According to Fig. 5, we can denote the polarization distribution of the DFWM signal across the beam image as the arrows show in Figs. 6(a) and 6(c). Then, we can discuss the DFWM signal intensity under various polarization directions. It is observed that the DFWM signal intensity is space-variant across the signal image. The DFWM signal gets highest when
Figure 6.Polarization distribution across the DFWM images, and the polarization-resolved spectra retrieved from the single DFWM signal when (a) and (b)
In this manner, the polarization-resolved spectra can be retrieved from a single DFWM signal image. As shown in Figs. 6(a) and 6(c), we denoted the vertical direction on the DFWM signal images as 0° and segmented the images radially every 15°. Then, we integrated the intensity along the straight lines. Finally, the integrated intensity was plotted with respect to the angle, and the polarization-resolved spectra were thus obtained [Figs. 6(b) and 6(d)]. It is seen that the DFWM signal attains a maximum at
5. CONCLUSION
In this work, we realized the single-scan polarization-resolved DFWM spectroscopy in the Rb atomic medium based on the space-variant polarization characteristics of the vector beam. In the experimental scheme, a vector beam is employed as the probe beam, and the two pump beams remain linearly polarized. As the polarization and intensity of the DFWM signal are closely dependent on the polarization state of the probe beam when the pump beams are kept invariant, a vector probe beam with space-variant states of polarization is able to generate a DFWM signal with space-variant states of polarization and intensity. Therefore, the polarization and intensity information can be retrieved from the single DFWM signal image. Compared with the traditional shot-to-shot polarization-resolved spectroscopy, the single-scan polarization-resolved spectrum is of particular importance in studying the samples of poor light stability and fast optical processes. In addition, the scheme proposed in this work based on the vector field is simple to use. To the best of our knowledge, this is the first time that single-scan polarization-resolved spectrum detection has been realized based on the vector field. This work not only provides a simple but efficient single-scan polarization-resolved DFWM method but also blazes a path for designing other single-scan polarization-resolved spectral or imaging methods, which would be of special significance for the samples of poor light stability and fast optical processes. In the next step, we will apply the single-scan polarization-resolved DFWM method to studying the micro-structure of the biological samples of poor light stability.
References
[1] A. J. Brown. Spectral curve fitting for automatic hyperspectral data analysis. IEEE Trans. Geosci. Remote Sens., 44, 1601-1608(2006).
[2] A. J. Brown. Equivalence relations and symmetries for laboratory, LIDAR, and planetary Müeller matrix scattering geometries. J. Opt. Soc. Am. A, 31, 2789-2794(2014).
[3] A. J. Brown, T. I. Michaels, S. Byrne, W. Sun, T. N. Titus, A. Colaprete, M. J. Wolff, G. Videen, C. J. Grund. The case for a modern multiwavelength, polarization-sensitive LIDAR in orbit around mars. J. Quant. Spectrosc. Radiat. Transfer, 153, 131-143(2015).
[4] N. Gisin, G. Ribordy, W. Tittel, H. Zbinden. Quantum cryptography. Rev. Mod. Phys., 74, 145-195(2002).
[5] A. E. Bracamonte, P. H. Vaccaro. Dissection of rovibronic band structure by polarization-resolved degenerate four-wave mixing spectroscopy. J. Chem. Phys., 119, 887-901(2003).
[6] Z. Liu, Z. Wang, X. Wang, X. Xu, X. Chen, J. Cheng, X. Li, S. Chen, J. Xin, S. T. C. Wong. Fiber bundle based probe with polarization for coherent anti-Stokes Raman scattering microendoscopy imaging. Proc. SPIE, 8588, 85880F(2013).
[7] A. M. Zheltikov, N. I. Koroteev, A. N. Naumov, V. N. Ochkin, S. Y. Savinov, S. N. Tskhai. Measurement of electric fields in a plasma with the aid of the coherent four-wave mixing polarisation technique. Quantum Electron., 29, 73-76(1999).
[8] Y. Prior. Three-dimensional phase matching in four-wave mixing. Appl. Opt., 19, 1741-1743(1980).
[9] G. Knopp, P. P. Radi, T. Gerber. Dispersed fs-FWM for investigations of low frequency vibrations of transient species in combustion. Chimia, 65, 339-341(2011).
[10] X. Cheng, Z. Ren, J. Wang, Y. Miao, X. Xu, L. Jia, H. Fan, J. Bai. Quantitative measurement of rubidium isotope ratio using forward degenerate four-wave mixing. Spectrochim. Acta B Atom. Spectros., 70, 39-44(2012).
[11] R. E. Compton. Nonlinear optics in non-equilibrium microplasmas(2011).
[12] S. Adachi, Y. Takagi, J. Takeda, K. A. Nelson. Optical sampling four-wave-mixing experiment for exciton relaxation processes. Opt. Commun., 174, 291-298(2000).
[13] S. Ye, S. Ma, F. Wei, H. Li. Intramolecular vibrational coupling in water molecules revealed by compatible multiple nonlinear vibrational spectroscopic measurements. Analyst, 137, 4981-4987(2012).
[14] C. Krafft, B. Dietzek, J. Popp. Raman and CARS microspectroscopy of cells and tissues. Analyst, 134, 1046-1057(2009).
[15] F. Munhoz, H. Rigneault, S. Brasselet. Polarization-resolved four-wave mixing microscopy for structural imaging in thick tissues. J. Opt. Soc. Am. B, 29, 1541-1550(2012).
[16] I. V. Fedotov, P. A. Zhokhov, A. B. Fedotov, A. M. Zheltikov. Probing the ultrafast nonlinear-optical response of ionized atmospheric air by polarization-resolved four-wave mixing. Phys. Rev. A, 80, 015802(2009).
[17] J. Tao, Z. Dong, J. K. W. Yang, Q. J. Wang. Plasmon excitation on flat graphene by s-polarized beams using four-wave mixing. Opt. Express, 23, 7809-7819(2015).
[18] R. Paiella, G. Hunziker, K. J. Vahala, U. Koren. Measurement of the interwell carrier transport lifetime in multiquantum-well optical amplifiers by polarization-resolved four-wave mixing. Appl. Phys. Lett., 69, 4142-4144(1996).
[19] F. Masia, W. Langbein, P. Borri. Polarization-resolved ultrafast dynamics of the complex polarizability in single gold nanoparticles. Phys. Chem. Chem. Phys., 15, 4226-4232(2013).
[20] J. Ishi-Hayase, K. Akahane, N. Yamamoto, M. Kujiraoka, J. Inoue, K. Ema, M. Tsuchiya, M. Sasaki. Coherent dynamics of excitons in a stack of self-assembled InAs quantum dots at 1.5-μm waveband. J. Lumin., 119–120, 318-322(2006).
[21] A. Shalit, Y. Prior. Time resolved polarization dependent single shot four wave mixing. Phys. Chem. Chem. Phys., 14, 13989-13996(2012).
[22] Y. Kozawa, S. Sato. Optical trapping of micrometer-sized dielectric particles by cylindrical vector beams. Opt. Express, 18, 10828-10833(2010).
[23] D. P. Biss, K. S. Youngworth, T. G. Brown. Dark-field imaging with cylindrical-vector beams. Appl. Opt., 45, 470-479(2006).
[24] G. Milione, T. A. Nguyen, J. Leach, D. A. Nolan, R. R. Alfano. Using the nonseparability of vector beams to encode information for optical communication. Opt. Lett., 40, 4887-4890(2015).
[25] J. Wang, X. Yang, Z. Dou, S. Qiu, J. Liu, Y. Chen, M. Cao, H. Chen, D. Wei, K. Müller-Dethlefs, H. Gao, F. Li. Directly extracting the authentic basis of cylindrical vector beams by a pump-probe technique in an atomic vapor. Appl. Phys. Lett., 115, 221101(2019).
[26] Y. Chen, F. Wang, L. Liu, C. Zhao, Y. Cai, O. Korotkova. Generation and propagation of a partially coherent vector beam with special correlation functions. Phys. Rev. A, 89, 013801(2014).
[27] J. Che, P. Zhao, D. Ma, Y. Zhang. Kerr-nonlinearity-modulated dressed vortex four-wave mixing from photonic band gap. Opt. Express, 28, 18343-18350(2020).
[28] J. Wang, X. Yang, Y. Li, Y. Chen, M. Cao, D. Wei, H. Gao, F. Li. Optically spatial information selection with hybridly polarized beam in atomic vapor. Photon. Res., 6, 451-456(2018).
[29] T. Jiao, X. Cheng, Q. Zhang, W. Li, Z. Ren. Multi-wave mixing using a single vector optical field. Appl. Phys. Lett., 115, 201104(2019).
[30] S. C. McEldowney, D. M. Shemo, R. A. Chipman. Vortex retarders produced from photo-aligned liquid crystal polymers. Opt. Express, 16, 7295-7308(2008).
[31] D. A. Steck. Rubidium 87 D line data(2021).
[32] R. W. Boyd. Nonlinear Optics(2002).
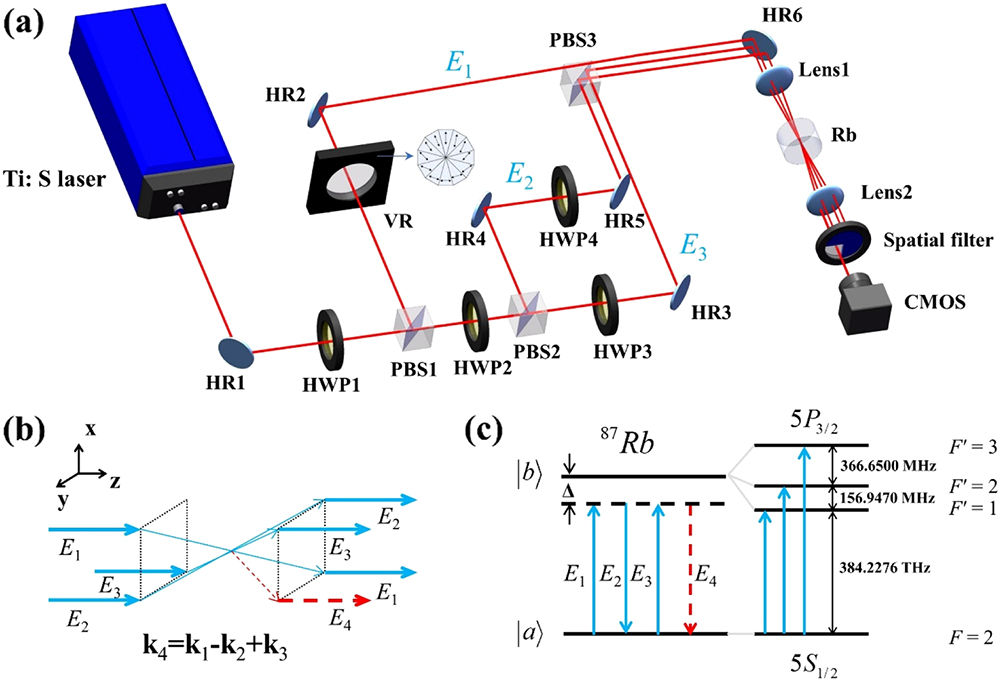
Set citation alerts for the article
Please enter your email address