
- Photonics Research
- Vol. 10, Issue 4, 913 (2022)
Abstract
1. INTRODUCTION
Quantum dots (QDs) are an important low-dimensional semiconductor material whose sizes in three dimensions are no more than twice the exciton Bohr radius of the corresponding semiconductor material. When QDs interact with their solid-state environment, we can obtain a light-atom continuum with excitonic Rabi rotation [1–4]. CdSe/ZnS as a kind of colloidal quantum dot (CQD) has been used to investigate photoluminescence (PL) blinking, power-law behavior, and single photon sources [5–7]. CdSe nanocrystal is a basic kind of CQD [8].
The nonlinear optical phenomena caused by placing CQDs in optical microcavities are worthy of attention. When strong coupling occurs, the coupling between the light and matter will produce new mixing states, which is the basis of quantum information systems such as quantum entanglement, quantum phase gates, ultralow power switches, and lasers [9–11]. Most of the achievements for the cavity quantum electrodynamics require low temperatures [12] and complex manufacturing methods [13]. To achieve strong coupling at room temperature, the conditions are more severe. When the loss rate of the cavity and the decay rate of the oscillators are not enough to offset the coupling strength between two oscillators with the same energy, the vacuum Rabi splitting will occur [14].
Recently, it has been reported that using 2D semiconductors and introducing a gain material embedded dielectric optical microcavity structure to study the strong coupling phenomenon at room temperature helps to obtain a Rabi splitting of about 36.0 meV [15]. There are also reports that researchers demonstrated the strong coupling between CdSe nanoplatelets and an open planar microcavity at room temperature, in which vacuum Rabi splitting of 66.0 meV was observed [16]. In addition, the surface plasmon resonator can satisfy a sufficiently small mode volume, and a strong coupling phenomenon can be observed [17]. These are all through scattering measurement research. However, strong coupling phenomena are sometimes difficult to interpret [18] because they are inevitably affected by other effects, such as enhanced absorption [19], Fano resonances in the weak coupling regime [20,21], or various polarization and symmetry artifacts. Therefore, only the scattering measurement is performed, and the evidence for characterizing strong coupling is not very convincing. But phenomena such as Fano interference cannot interfere with the study of PL spectroscopy; the splitting of the PL spectrum determinedly represents the generation of the phenomenon of vacuum Rabi splitting [12]. In larger systems, like metal films and Fabry–Perot (F–P) interferometers, the researchers usually could only see the report for the spectrum of the lower polariton, because the upper polariton (UP) will turn to an uncoupled incoherent state due to a very fast lifetime [22–25]. Here, we creatively use a hybrid microstructure with a low–high–low refractive index to achieve strong coupling between CQDs and a microcavity at room temperature. In our experiments, PL spectral splitting could be observed on the ensemble level, and the transition of the UP was observed.
Sign up for Photonics Research TOC. Get the latest issue of Photonics Research delivered right to you!Sign up now
At the same time, in our hybrid refractive-index waveguide, bound states can exist above the potential barrier. The existence of this phenomenon, the so-called bound state in the continuum (BIC) has been proven [26]. There have been some researches about BIC on photoelectronic structures [27–30]. The application of BIC in waveguide structures with different refractive-index distributions has also been reported [31,32]. Under specific waveguide structure parameters, the leakage channels have a destructive interference, resulting in a lower optical loss. In this study, the photonic quasi-BIC in our special refractive-index waveguide was investigated. The PL spectral spitting was attributed to the strong coupling between F–P BIC and the exciton of the CQDs on the surface of the polymer-SOI waveguide.
2. EXPERIMENT
A. Cavity with BICs
In the experiment, a Si waveguide with a height of 340 nm and varying width of
Figure 1.Quasi-BIC in hybrid refractive-index waveguide. (a) Schematic diagram of hybrid waveguide. The etched SOI is 340 nm thick with a gradually varying width
In our low–high–low refractive-index hybrid structure, in the vertical direction, the silicon with a high refractive index acts as a reflecting mirror at the bottom, and the upper surface of the low refractive-index material acts as the top mirror to form the vertical SU8-SOI F–P cavity. On the other hand, when the width of the waveguide is suitable, the quasi-BIC is formed based on the photon-bound mechanism. This is because destructive interference occurs between various dissipative channels, leading to the loss of light being greatly reduced. In our hybrid refractive-index waveguide, the effective refractive index of the waveguide region [as shown in Fig. 1(b)] is higher than that of other regions, so a potential well can be effectively formed. Obviously, the light can be confined in this potential well. The refractive index of the upper layer (SU8) is smaller than the refractive index of the lower waveguide material (Si). We know that the electric potential is inversely proportional to the dielectric refractive index. The potential well of the vertical polarization mode should be higher than that of the horizontal polarization mode. By solving the light propagation equation [31] with a wavelength of 655 nm, the potential of the hybrid waveguide with width
The Hamiltonian for this process can be expressed as [31]
Here, integer
B. Strong Coupling between CQDs and F-P BIC
Next, we exploited the F–P BIC for strong coupling. Using a calibrated pipette, 0.5 μL CQDs in an N-hexane solvent with a concentration of
Figure 2.Schematic diagram of waveguide structure and coupled CQD energy level. (a) CQDs were drop-casted onto the polymer waveguide surface. (b) Energy-level structure of the CQD-cavity system with large coupling strength. When strong coupling occurs, the exciton energy level G is split into two sub-energy levels.
When the CQDs are strongly coupled with the optical microcavity, the system has an energy level structure, as shown in Fig. 2(b) [35,36]. The exciton energy level G will split into two different energy levels with a spacing of
First, we measured the PL spectrum of CQDs at different positions on the waveguide. The results are shown in Figs. 3(a)–3(f), and the waveguide widths are, respectively,
Figure 3.PL spectra of CQDs at different widths on the waveguide, from (a) to (f), and the waveguide widths from
In the region with an appropriate width
We measured the reflection (RL) spectrum of the cavity without CQDs, as shown in Fig. 3(c) with blue curve.
The relative intensity of PL spectra generated by the LP transition in Fig. 3(c) is higher than that of the UP transition. At this point, there are more carriers at the
The strong coupling between CQDs and cavities led to the splitting of the PL spectra, which depended on the collection angle. The PL spectrum variation of CQDs with the divergence angle of emission could be collected in the experiment. We used a spectrometer with a sensitive CCD to measure the spectral image over a period of time. A raw angle-resolved spectral image with a maximum collection angle of 36.9° could be measured using the microscope objective (
As shown in Fig. 4(a), the brightness of the measured spectral image represents the relative strength of the PL spectrum, the horizontal axis represents the wavelength, and the vertical axis corresponds to the collection angle, which is symmetric at both ends and is centered at zero degrees. The CQDs spectrum corresponds to the width
Figure 4.Spectral image of CQDs on the waveguide with width
Figure 5.Strong coupling between CQDs and SU8-SOI waveguide. At different angles, the peak energies of the PL split spectra change with the angle: (a) at waveguide width
For this system, the eigenvalues can be expressed as [37]
The optical cavity formed by the low–high–low refractive-index structure is very important. To prove this, we measured the PL spectrum of CQDs with a concentration of
3. DISCUSSION
We observed strong coupling of the CQDs associated with the F–P BIC of the cavity based on a variation-width waveguide. Benefiting from the localization effect of the quasi-BIC mode on the light field, the eigenmode of the cavity can resonate with the exciton transition of the CQDs, resulting in Rabi splitting, which is manifested as the splitting phenomenon of the PL spectrum. It is a strong coupling between the CQDs and the cavity. The
That is why we can only observe strong coupling with a certain waveguide width because, as mentioned above, quasi-BIC can only be formed when the waveguide width is appropriate. For example, in some width regions of the waveguide, the coupling strength between the CQDs and the F–P cavity is not high; therefore, the spectral splitting is not obvious. The peak energies varying with the angle are shown in Fig. 5(c), which corresponds to the spectrum in Fig. 3(a) (
As for the bigger widths, the TE mode supports multiple modes in the CQDs’ spectral range. These modes and the F–P BIC mode in the vertical direction jointly act on the exciton emission of CQDs, causing the PL spectrum to produce more peaks, as shown in Figs. 3(e) and 3(f). For width
However, as shown in Fig. 5(b), the PL spectrum at 11.0° also shows peaks at 1.846 eV and 1.943 eV, and the widths of the spectrum are 16.5 meV and 14.1 meV, respectively. In fact, this is due to the Purcell effect caused by the weak coupling between the CQDs and the microcavity, which results in the enhanced luminescence of the CQDs. At the same energy position, the RL spectrum also has the spectral peak, indicating that there is a microcavity mode. As shown in Fig. 5(b), the RL peaks are located at 1.840 eV and 1.942 eV, which are very close to the peaks of the PL spectrum. In the higher- and lower-energy regions, the variation of the energies of these two peaks with angle is also shown in Fig. 5(d) (RL with cross symbols, PL with circles). The RL and PL spectral peaks appear at very close energy positions with the same changing trend, which shows the weak coupling between the CQDs and the cavity modes. The RL and PL spectra have a slight deviation in position, which may be due to the high concentration of quantum dots that possibly form a thin film on top of the SU8 with a higher refractive index than air. Through simulation, it is found that the CQDs layer will not have a great impact on our model. Therefore, we did not take into account the CQDs in modeling.
To sum up, in the hybrid material with a low–high–low refractive index, the strong coupling was generated between the CQDs and F–P BIC at room temperature. We have observed Rabi splitting due to strong coupling by measuring the PL spectrum. On a single layer material with a low refractive index (
4. CONCLUSION
In conclusion, we fabricated SOI-material waveguides with varying widths. The hybrid low–high–low waveguide was formed by coating the SU8 polymer with a low refractive index (compared to silicon) onto the SOI. The special structure formed F–P BIC. Due to the destructive interference between different loss channels, the optical field is localized in the waveguide. The PL spectra of CQDs on the waveguides were measured and analyzed at different acquisition angles. Because of the strong coupling between the exciton of CQDs and the cavity modes, the PL spectrum split into two or more peaks. Strong coupling was generated between CQDs and F–P BIC, and the Rabi splitting energy reached 96.0 meV. This provides a new idea for the future realization of quantum integrated circuits and the construction of a quantum optical experimental platform.
Acknowledgment
Acknowledgment. Projects were supported by the National Natural Science Foundation of China, the Strategic Priority Research Program (A) of Chinese Academy of Sciences, and the National Key Research and Development Program of China.
References
[1] A. Zrenner, E. Beham, S. Stufler, F. Findeis, M. Bichler, G. Abstreiter. Coherent properties of a two-level system based on a quantum-dot photodiode. Nature, 418, 612-614(2002).
[2] S. Stufler, P. Ester, A. Zrenner, M. Bichler. Quantum optical properties of a single In
[3] T. Guenther, C. Lienau, T. Elsaesser, M. Glanemann, V. M. Axt, T. Kuhn, S. Eshlaghi, A. D. Wieck. Erratum: coherent nonlinear optical response of single quantum dots studied by ultrafast near-field spectroscopy. Phys. Rev. Lett., 89, 179901(2002).
[4] H. Takagi, T. Nakaoka, K. Watanabe, N. Kumagai, Y. Arakawa. Coherently driven semiconductor quantum dot at a telecommunication wavelength. Opt. Express, 16, 13949-13954(2008).
[5] A. L. Efros, M. Rosen. Random telegraph signal in the photoluminescence intensity of a single quantum dot. Phys. Rev. Lett., 78, 1110-1113(1997).
[6] C. Galland, Y. Ghosh, A. Steinbrück, M. Sykora, J. A. Hollingsworth, V. I. Klimov, H. Htoon. Two types of luminescence blinking revealed by spectroelectrochemistry of single quantum dots. Nature, 479, 203-207(2011).
[7] M. Kuno, D. P. Fromm, H. F. Hamann, A. Gallagher, D. J. Nesbitt. Nonexponential ‘blinking’ kinetics of single CdSe quantum dots: a universal power law behavior. J. Chem. Phys., 112, 3117-3120(2000).
[8] H. Gross, J. M. Hamm, T. Tufarelli, O. Hess, B. Hecht. Near-field strong coupling of single quantum dots. Sci. Adv., 4, eaar4906(2018).
[9] Y. Sato, Y. Tanaka, J. Upham, Y. Takahashi, T. Asano, S. Noda. Strong coupling between distant photonic nanocavities and its dynamic control. Nat. Photonics, 6, 56-61(2012).
[10] J. D. Thompson, T. G. Tiecke, N. P. de Leon, J. Feist, A. V. Akimov, M. Gullans, A. S. Zibrov, V. Vuletic, M. D. Lukin. Coupling a single trapped atom to a nanoscale optical cavity. Science, 340, 1202-1205(2013).
[11] M. Keller, B. Lange, K. Hayasaka, W. Lange, H. Walther. Deterministic coupling of single ions to an optical cavity. Appl. Phys. B, 76, 125-128(2003).
[12] H. X. Leng, B. Szychowski, M. C. Daniel, M. Pelton. Strong coupling and induced transparency at room temperature with single quantum dots and gap plasmons. Nat. Commun., 9, 4012(2018).
[13] J. Vuckovic, Y. Yamamoto. Photonic crystal microcavities for cavity quantum electrodynamics with a single quantum dot. Appl. Phys. Lett., 82, 2374-2376(2003).
[14] G. Khitrova, H. M. Gibbs, M. Kira, S. W. Koch, A. Scherer. Vacuum Rabi splitting in semiconductors. Nat. Phys., 2, 81-90(2006).
[15] X. C. Zhao, Y. H. Yan, Z. Z. Cui, F. Liu, S. W. Wang, L. X. Sun, Y. W. Chen, W. Lu. Realization of strong coupling between 2D excitons and cavity photons at room temperature. Opt. Lett., 45, 6571-6574(2020).
[16] L. C. Flatten, S. Christodoulou, R. K. Patel, A. Buccheri, D. M. Coles, B. P. L. Reid, R. A. Taylor, I. Moreels, J. M. Smith. Strong exciton–photon coupling with colloidal nanoplatelets in an open microcavity. Nano Lett., 16, 7137-7141(2016).
[17] R. Chikkaraddy, B. de Nijs, F. Benz, S. J. Barrow, O. A. Scherman, E. Rosta, A. Demetriadou, P. Fox, O. Hess, J. J. Baumberg. Single-molecule strong coupling at room temperature in plasmonic nanocavities. Nature, 535, 127-130(2016).
[18] M. Wersäll, J. Cuadra, T. J. Antosiewicz, S. Balci, T. Shegai. Observation of mode splitting in photoluminescence of individual plasmonic nanoparticles strongly coupled to molecular excitons. Nano Lett., 17, 551-558(2017).
[19] G. L. Liu, Y.-T. Long, Y. Choi, T. Kang, L. P. Lee. Quantized plasmon quenching dips nanospectroscopy via plasmon resonance energy transfer. Nat. Methods, 4, 1015-1017(2007).
[20] X. Wu, S. K. Gray, M. Pelton. Quantum-dot-induced transparency in a nanoscale plasmonic resonator. Opt. Express, 18, 23633-23645(2010).
[21] Z. J. Yang, T. J. Antosiewicz, T. Shegai. Role of material loss and mode volume of plasmonic nanocavities for strong plasmon-exciton interactions. Opt. Express, 24, 20373-20381(2016).
[22] J. Bellessa, C. Bonnand, J. C. Plenet, J. Mugnier. Strong coupling between surface plasmons and excitons in an organic semiconductor. Phys. Rev. Lett., 93, 036404(2004).
[23] J. R. Tischler, M. S. Bradley, V. Bulović, J. H. Song, A. Nurmikko. Strong coupling in a microcavity LED. Phys. Rev. Lett., 95, 036401(2005).
[24] V. M. Agranovich, M. Litinskaia, D. G. Lidzey. Cavity polaritons in microcavities containing disordered organic semiconductors. Phys. Rev. B, 67, 085311(2003).
[25] T. Schwartz, J. A. Hutchison, J. Léonard, C. Genet, S. Haacke, T. W. Ebbesen. Polariton dynamics under strong light–molecule coupling. ChemPhysChem, 14, 125-131(2013).
[26] J. V. Neumann, E. Wigner. On some peculiar discrete eigenvalues. Phys. Z., 30, 465-467(1929).
[27] X. Gao, B. Zhen, M. Soljačić, H. Chen, C. W. Hsu. Bound states in the continuum in fiber Bragg gratings. ACS Photon., 6, 2996-3002(2019).
[28] D. C. Marinica, A. G. Borisov, S. V. Shabanov. Bound states in the continuum in photonics. Phys. Rev. Lett., 100, 183902(2008).
[29] C. W. Hsu, B. Zhen, J. Lee, S.-L. Chua, S. G. Johnson, J. D. Joannopoulos, M. Soljačić. Observation of trapped light within the radiation continuum. Nature, 499, 188-191(2013).
[30] A. Kodigala, T. Lepetit, Q. Gu, B. Bahari, Y. Fainman, B. Kanté. Lasing action from photonic bound states in continuum. Nature, 541, 196-199(2017).
[31] C. Zou, J. Cui, F. Sun, X. Xiong, X. Zou, Z. Han, G. Guo. Guiding light through optical bound states in the continuum for ultrahigh-
[32] X. Xu, S. Jin. Strong coupling of single quantum dots with low-refractive-index/high-refractive-index materials at room temperature. Sci. Adv., 6, eabb3095(2020).
[33] Z. Yu, X. Xi, J. Ma, H. K. Tsang, C. Zou, X. Sun. Photonic integrated circuits with bound states in the continuum. Optica, 6, 1342-1348(2019).
[34] Thermo Fisher. The Molecular Probes Handbook, Qdot Nanocrystals, Section 6.6.
[35] T. H. Stievater, X. Li, D. G. Steel, D. Gammon, D. S. Katzer, D. Park, C. Piermarocchi, L. J. Sham. Rabi oscillations of excitons in single quantum dots. Phys. Rev. Lett., 87, 133603(2001).
[36] A. L. Efros, M. Rosen, M. Kuno, M. Nirmal, D. J. Norris, M. Bawendi. Band-edge exciton in quantum dots of semiconductors with a degenerate valence band: dark and bright exciton states. Phys. Rev. B, 54, 4843-4856(1996).
[37] J. Wiersig. Formation of long-lived, scarlike modes near avoided resonance crossings in optical microcavities. Phys. Rev. Lett., 97, 253901(2006).
[38] J. P. Reithmaier, G. Sek, A. Löffler, C. Hofmann, S. Kuhn, S. Reitzenstein, L. V. Keldysh, V. D. Kulakovskii, T. L. Reinecke, A. Forchel. Strong coupling in a single quantum dot-semiconductor microcavity system. Nature, 432, 197-200(2004).
[39] E. Melik-Gaykazyan, K. Koshelev, J. H. Choi, S. S. Kruk, A. Bogdanov, H. G. Park, Y. Kivshar. From Fano to quasi-BIC resonances in individual dielectric nanoantennas. Nano Lett., 21, 1765-1771(2021).
[40] K. Koshelev, S. Kruk, E. Melik-Gaykazyan, J. H. Choi, A. Bogdanov, H. G. Park, Y. Kivshar. Subwavelength dielectric resonators for nonlinear nanophotonics. Science, 367, 288-292(2020).
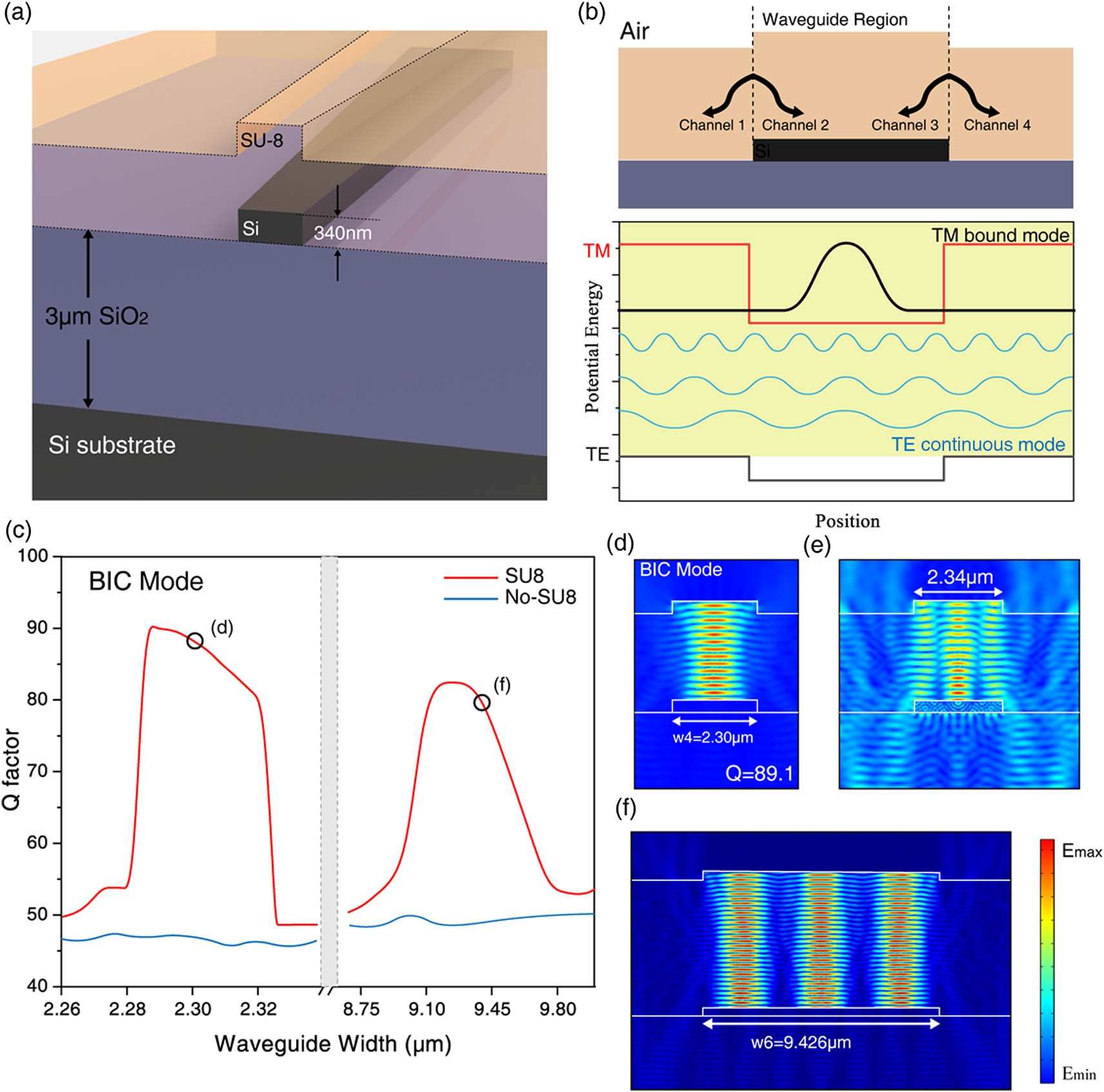
Set citation alerts for the article
Please enter your email address