Guanyu Chen, Nanxi Li, Jun Da Ng, Hong-Lin Lin, Yanyan Zhou, Yuan Hsing Fu, Lennon Yao Ting Lee, Yu Yu, Ai-Qun Liu, Aaron J. Danner, "Advances in lithium niobate photonics: development status and perspectives," Adv. Photon. 4, 034003 (2022)

Search by keywords or author
- Advanced Photonics
- Vol. 4, Issue 3, 034003 (2022)
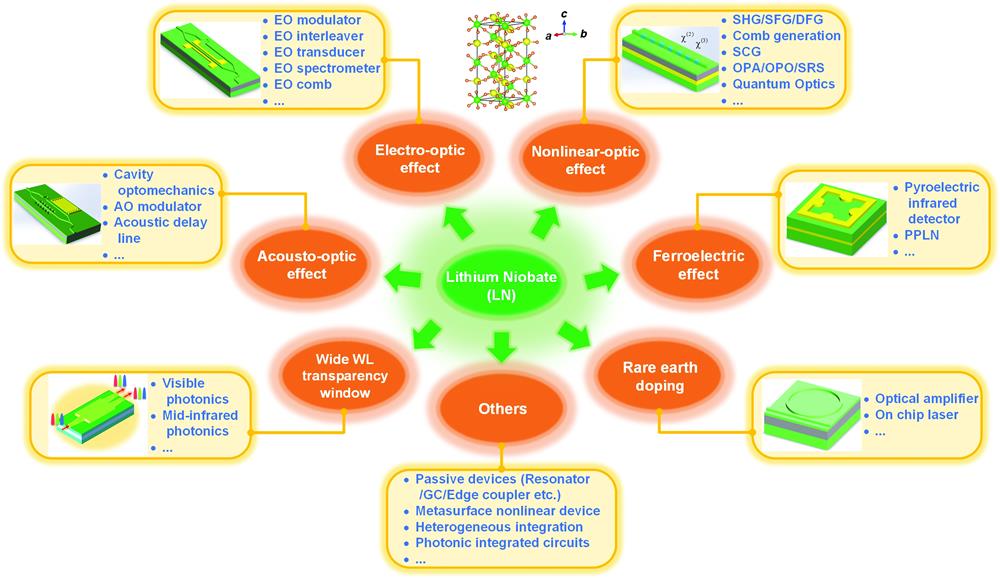
Fig. 1. Overview of LN photonics. Top middle inset is LN crystal structure. EO, electro-optic; SHG, second harmonic generation; SFG/DFG, sum/difference frequency generation; SCG, supercontinuum generation; OPA/OPO, optical parametric amplification/oscillation; SRS, stimulated Raman scattering; PPLN, periodically poled lithium niobate; GC, grating coupler; WL, wavelength; AO, acousto-optic.
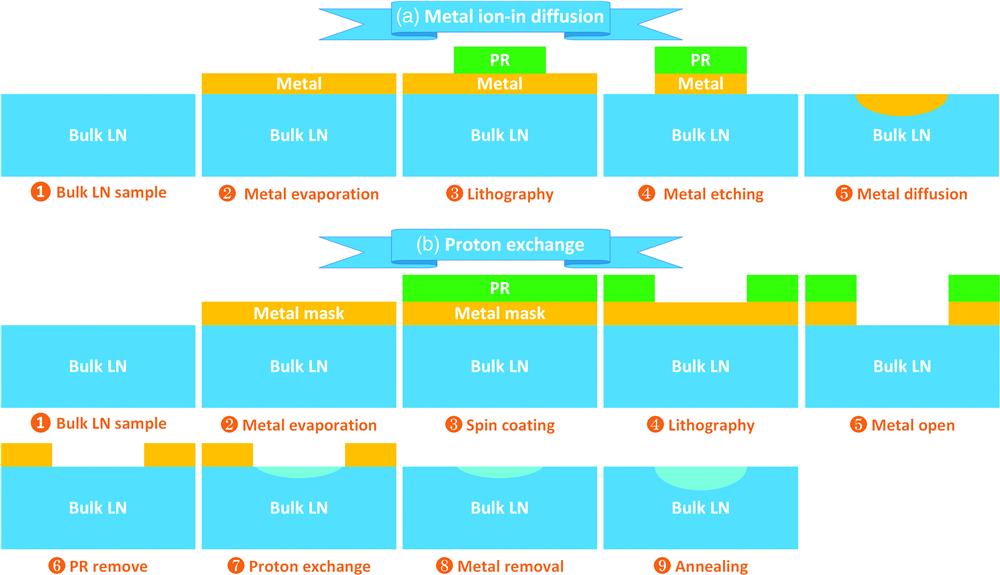
Fig. 2. Process flow of planar LN device fabrication. Illustration of (a) metal ion-in diffusion and (b) PE methods for planar photonic device fabrication in bulk LN crystals (dimensions are not drawn to scale). PR, photoresist.
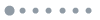
Fig. 3. Process flows of (a) CIS and (b) lapping and polishing technologies. Dimensions are not drawn to scale.
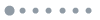
Fig. 4. Heterogeneous integrated LN devices. (a) Schematic structure, (b) optical, and (c) atomic force microscopic images of an LN on silica hybrid micro-resonator. (a)–(c) Adapted from Ref. 161 © 2015 Wiley-VCH Verlag GmbH and Co. (d) 3D schematic structure, (e) cross section and optical field distribution of a on LN hybrid MZI modulator. (d) and (e) Adapted from Ref. 169; all article content, except where otherwise noted, is licensed under a Creative Commons Attribution (CC BY) license.
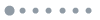
Fig. 5. Dry etching results of LN. (a) SEM image of the LN cross section and (b) current changed along etching depth in end point detection after based etching. (a) and (b) Adapted from Ref. 182 © 2008 American Institute of Physics (AIP). (c) SEM, (d) AFM, and (e) XPS images of LN sample after Ar-based dry etching. (c)–(e) Adapted with permission from Ref. 175.
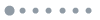
Fig. 6. Wet etching results of LN. SEM images of the LN etched cross section using undiluted (a) 0% and (b) 20% of adipic acid, (c) 0% and (d) 20% of adipic acid with 0.6% of lithium benzoate, (e) 20% and (f) 30% of adipic acid with 0.3% of lithium carbonate (concentrations in percent represent mole fractions). (a)–(f) Adapted from Ref. 198 © 2006 Wiley Periodicals, Inc. (g) SEM image of photonic crystals (PhCs) using ion implantation and wet etching. Adapted with permission from Ref. 138 © 2010 American Vacuum Society.
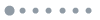
Fig. 7. LN-based microresonators. (a) Schematic experimental setup for characterizing a mechanical polishing bulk LN whispering-gallery resonator and its corresponding measured factor. Adapted from Ref. 227 © 2011 AIP. (b) Resonance spectra of the fabricated microdisk using ECR RIE technology in TFLN. Inset shows the microscope image of tapered fiber coupling on top of the device. Zoom in views are the details of representative resonance dips. Adapted with permission from Ref. 228 © 2014 Optical Society of America (OSA). (c) SEM (top) and microscopic images (bottom) of microring and microracetrack ring with various lengths, and (d) its measured transmission spectrum. (c) and (d) Adapted with permission from Ref. 192 © 2017 OSA. (e) Microscope image of the waveguide coupled TFLN microring and (f) its measured transmission spectrum. The Q factors for (g) TE and (h) TM modes fitted by Lorentz-shape curves. (e)–(h) Adapted with permission from Ref. 224 © 2022 Chinese Optical Society (COS).
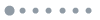
Fig. 8. LN-based GCs. (a) Schematic structure, (b) simulated electric field distribution and (c) measured transmission spectrum of 1D chirped GC in TFLN. (a)–(c) Adapted with permission from Ref. 259 © 2020 OSA. (d) Schematic structure of a 2D GC in TFLN. Measured and simulated (e) transmission spectra and (f) polarization dependence loss of the TFLN 2D GC. (d)–(f) Adapted with permission from Ref. 265 © 2021 OSA.
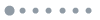
Fig. 9. LN-based edge coupler. (a) Schematic structure of the bilayer edge coupler and its corresponding mode profiles at different positions. (b) Simulated and measured coupling efficiency versus different tip widths in the tapered slab region. (c) Additional insertion loss with respect to coupling misalignment (TE mode). (a)–(c) Adapted with permission from Ref. 246 © 2019 OSA.
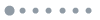
Fig. 10. TFLN EO tunable microring resonator. (a) Schematic structure (top), cross section (bottom left), and SEM images of the Z-cut TFLN microring modulator, and (b) its EO resonance shift curve. (a) and (b) Adapted with permission from Ref. 181 © 2007 Nature Publishing Group.
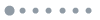
Fig. 11. TFLN-based modulators. (a) Microscopic image of TFLN MZI modulator (inset is its schematic cross section). (b) Measured transmission spectrum of a 2-cm long device. (c) Measured high speed data transmission results of NRZ, 4-ASK, and 8-ASK signals. (a)–(c) Adapted with permission from Ref. 31 © 2018 Springer Nature Limited (SNL). (d) SEM images (top: full SEM image; bottom: zoom-in image of the PhC details). (e) Schematic structure of the TFLN PhC modulator. (d) and (e) Adapted with permission from Ref. 241. (f) Schematic structure of the MIM, and insets are cross section mode profiles at different positions. Adapted from Ref. 276. (g) Schematic structure of the TFLN DBR modulator, and SEM images of the (h) DBR and (i) modulation region. (g)–(i) Adapted with permission from Ref. 242 © 2021 COS. (j) Schematic structure and (k) measured curves of the TFLN-based DP-IQ modulator. X and Y represent two orthogonal polarization states, I and Q represent in-phase and quadrature branches. (j) and (k) Adapted with permission from Ref. 294 © 2022 Optica.
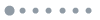
Fig. 12. EO tunable interleaver in TFLN. (a) Schematic structure of TFLN waveguide interleaver, and its measured tunable transmission spectra for (b) TE and (c) TM polarized input light. (a)–(c) Adapted with permission from Ref. 297 © 2018 OSA.
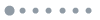
Fig. 13. TFLN-based EO devices for optical frequency controlling. (a) False colored SEM image of an EO tunable coupled microring resonator. (b) The programmable photonic molecule consists of a pair of identical coupled rings (resonant frequency ). Such a system has two distinct energy levels: symmetric (blue/blue shading) and antisymmetric (red/blue) optical modes are spatially out of phase by . The microwave field interacts with the two-level system through the large EO effect of TFLN. (a) and (b) Adapted with permission from Ref. 298 © The Author(s), under exclusive license to SNL 2018. (c) SEM image of a reconfigurable electro-optic frequency shifter. (d) Upshift and (e) downshift under 12.5 GHz microwave frequency and at 1601.2 nm wavelength ( ) show measured 80% CE and >0.99 shift ratio (defined as the ratio of the output power at the shifted frequency and the output power inside the bus waveguide). Inset shows the directions of energy flow and the spectra in dB scale. (c)–(e) Adapted with permission from Ref. 299 © The Author(s), under exclusive license to SNL 2021.
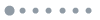
Fig. 14. EO-based microwave to optical transducer in TFLN. (a) Microscopic image of a TFLN-based transducer, and (b) its corresponding measured maximum transduction efficiency with respect to optical pump powers. (a) and (b) Adapted with permission from Ref. 300 © 2020 OSA. (c) Microscopic image of a triply resonant LN on sapphire transducer (zoom in: device details), and (d) its measured photon count rate versus microwave drive frequency with respect to different input microwave powers. (c) and (d) Adapted with permission from Ref. 301 © 2020 OSA. (e) Schematic of an EO converter in TFLN based on two coupled microring resonators (red) and a cointegrated superconducting resonator (yellow). DC bias is applied for optical mode tuning. (f) False color SEM image of the EO converter detail. Inset is the electric field distribution. (e) and (f) Adapted with permission from Ref. 302.
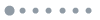
Fig. 15. EO waveguide spectrometer in TFLN. Microscopic image and device details of an EO TFLN waveguide spectrometer. Adapted with permission from Ref. 171 © The Author(s), under exclusive license to SNL 2019.
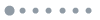
Fig. 16. LN-based nonlinear and quantum photonic devices. (a) Schematic structure of the TFPPLN microring. (b) False-color SEM images of the device cross section and coupling region detail. (c) Experimentally measured SHG power versus pump power. (a)–(c) Adapted with permission from Ref. 63 © 2019 OSA. (d) False color SEM image of TFLN PIC containing Kerr comb and EO add-drop filter. Adapted with permission from Ref. 76. (e) Measured transmission spectrum of the EO comb. Left inset shows a magnified view of several comb lines. Right inset shows measured transmission spectrum for several different modulation indices. Adapted with permission from Ref. 77 © The Author(s), under exclusive license to SNL 2019. (f) Measured transmission spectra with respect to different waveguide width. Adapted with permission from Ref. 79 © 2019 OSA. (g) Principle of OPA in dispersion engineered PPLN waveguide and simulated relative gain spectrum for three dispersion cases. Adapted with permission from Ref. 70 © 2022 OPTICA. (h) Schematic structure of the PPLN microring. Insets are the SEM images of the device details. Measured (i) PGR and (j) CAR. (h)–(j) Adapted with permission from Ref. 81 © 2020 American Physical Society (APS).
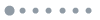
Fig. 17. Cavity optomechanics devices in LN. (a) Schematic of a band structure engineered surface acoustic resonator on TFLN. Inset is the microscopic image of the fabricated device. (b) Measured factor with respect to different resonator frequencies. (a) and (b) Adapted with permission from Ref. 311 © 2019 APS. Unit cell geometries of the (c) nanobeam optomechanical crystal and (d) 1D photonic shield. (e) SEM image of a 1D PhC cavity resonator for optomechanical mode generation. Left: full view of the device. Middle: top view of one device. Top right: top view of the 1D photonic shield region. Bottom right: SEM image of the nanobeam reflector coupling region. (c)–(e) Adapted with permission from Ref. 310 © 2019 OSA.
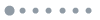
Fig. 18. Design of IDTs. Schematics of (a) straight and (b) concentric IDTs. (a) and (b) Adapted with permission from Ref. 315 © 2005 IEEE.
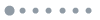
Fig. 19. LN-based AO modulators. Schematics of (a) MZI and (b) microring type AO modulators. (c) Cross section of the AO modulator. (a)–(c) Adapted with permission from Ref. 16 © 2019 Chinese Laser Press (CLP). (d) Microscopic image of a suspended AO MZI. (e) and spectra of microwave to optical conversion. The optical power detected by photodetector (PD) is 0.25 mW. (d) and (e) Adapted with permission from Ref. 17 © 2019 OSA. (f) Principal illustration of one AO modulator without resonator cavity. (g) Measured and spectra. (f) and (g) Adapted with permission from Ref. 312 © 2021 CLP. (h) Schematic of AO frequency shifter based on photonic BIC. Adapted with permission from Ref. 320 © 2021 American Chemical Society (ACS).
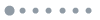
Fig. 20. LN-based ADL. (a) Microscopic image of the fabricated ADL. (b) Microscopic image of zoomed in view of the device. (c) Extracted IL and FBW of ADLs with respect to cell numbers. (a)–(c) Adapted with permission from Ref. 321 © 2018 IEEE.
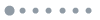
Fig. 21. Rare-earth-doped devices in LN. (a) Schematic structure of a doped TFLN device. Measured (b) photoluminescence spectra and (c) time-resolved photoluminescence in doped bulk LN and TFLN, respectively. (a)–(c) Adapted with permission from Ref. 94 © 2019 ACS. (d) Top left: SEM images of GC and microring patterned in TFLN. Top right: the stopping and range of ions in matter (SRIM) simulation of implantation depth distribution. Bottom: schematic electrical field distribution. (e) Transmission spectrum of a TFLN microring. (f) Measured fluorescence decay when the pumping frequency is detuned from the ring resonance. (d)–(f) Adapted from Ref. 95. (g) Schematic structure of an doped TFLN waveguide-based amplifier. Gain characterization with respect to different pump power when signal wavelength is (h) 1530 nm and (i) 1550 nm. (g)–(i) Adapted with permission from Ref. 33 © 2021 Wiley-VCH GmbH. (j) Signal power and (k) mode linewidth with respect to different pump powers. (j) and (k) Adapted with permission from Ref. 330 © 2021 OSA. (l) Modulated wavelength of microdisk laser with respect to pump power. Adapted with permission from Ref. 93 © 2021 OSA.
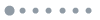
Fig. 22. TFLN-based pyroelectric infrared detector. (a) SEM image of the metamaterial top surface. (b) Schematic of the unit cell. (c) Microscopic image of the pyroelectric PD. (d) Measured detector response and optical absorption of pyroelectric PD with and without metamaterial structure. (a)–(d) Adapted with permission from Ref. 18 © 2017 OSA.
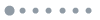
Fig. 23. Nonlinear metasurface devices in LN. (a) Schematic structure of TFLN metasurface for SHG. (b) Measured SHG power with respect to fundamental harmonic average power. (a) and (b) Adapted with permission from Ref. 341 © 2020 ACS. (c) Schematic structure of the diffraction mechanism in the metasurface. SEM images of the (d) full view metasurface (scale bar is ) and (e) zoom in nanopillars. (f) SHG CE of the diffraction orders with respect to pump power. (c)–(f) Adapted with permission from Ref. 342.
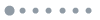
Fig. 24. TFLN modulator operated at visible wavelength range. (a) Microscopic image of the TFLN EO modulator. (b) Measured transmission spectrum and (c) curve. (a)–(c) Adapted with permission from Ref. 42 © 2019 OSA.
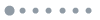
Fig. 25. Integrated SNSPD in LN. (a) Schematic structure of Ti diffused LN waveguide integrated with five in-line SNSPDs. Inset shows the detail of single SNSPD, which has length and 160 nm width. (b) Measured response time of an integrated SNSPD. (c) Measured signal and dark counts of the integrated SNSPD under different bias current. (a)–(c) Adapted with permission from Ref. 347. Published by IOP Publishing Ltd. (d) Top: schematic of a TFLN GC coupling light into an integrated U-shaped NbN SNSPD. Bottom left: device cross section. Bottom right: SEM image of the device detail. (e) Measured OCDE, (f) DCR and NEP with respect to for a long detector. , switching current; , bias current. (d)–(f) Adapted from Ref. 172. (g) Microscopic image of the on-chip integrated circuit containing one TFLN EO modulator and two NbTiN SNSPDs. (h) Measured count rates collected from the SNSPDs with a time tagging module (bottom) when EO modulator is driven with a ramp function with an amplitude of and frequency of 1 kHz (top). (g) and (h) Adapted with permission from Ref. 349.
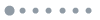
Fig. 26. Integrated Si PD onto LN passive circuit. (a) Schematic structure and (b) false colored SEM image of a TFLN waveguide with integrated Si PD. (a) and (b) Adapted from Ref. 34.
|
Table 1. Material property summary of LN.
|
Table 2. Summaries of metal ion-in diffusion method. T , temperature; TE, transverse electric; TM, transverse magnetic; N.A., not available/applicable; Zn, Zinc.
|
Table 3. Summary of heterogeneous integration of LN with other material systems. ALD, atomic layer deposition; N.A., not available/applicable; a-Si, amorphous silicon.
|
Table 4. Summary of LN dry etching technologies. PMMA, polymethyl methacrylate; HSQ, hydrogen silsesquioxane; MMA, methyl methacrylate; N.A., not available/applicable; RIE, reactive ion etching; ICP, inductively coupled plasma.
|
Table 5. Summary of LN-based EO modulators. HI, heterogeneous integration; DMT, discrete multitone; APE, annealed proton exchange; N.A., not available/applicable.
|
Table 6. Summary of LN-based devices for nonlinear and quantum photonic applications. MgLN, MgO-doped lithium niobate; ZnLN, Zn-doped lithium niobate; ZnOLN, ZnO-doped lithium niobate; PE, proton exchange; HI, heterogeneous integration; PIC, photonic integrated circuit; N.A., not available/applicable.
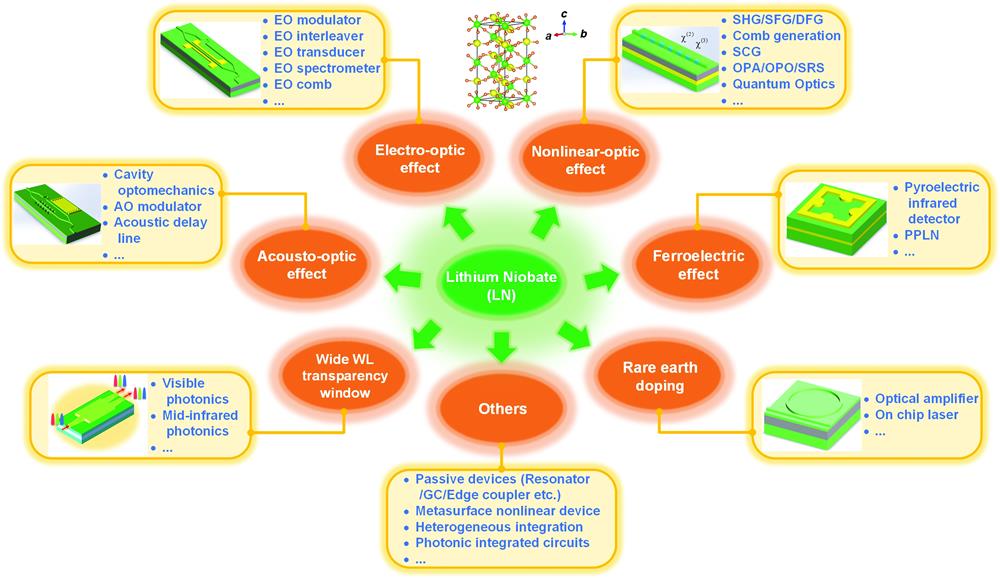
Set citation alerts for the article
Please enter your email address