
- Opto-Electronic Advances
- Vol. 4, Issue 9, 200092-1 (2021)
Abstract
Introduction
Optical metamaterial is composed of periodically arranged nano-optical elements whose size is much smaller than the working wavelength
When it comes to the manipulation of light for optical metasurfaces, generally speaking, there are two phase control approaches for optical metasurface: resonant phase and geometric phase, or called Pancharatnam-Berry phase
Look back over the past few decades, the research fields of metasurfaces have primarily been focused on classical regime, and enormous efforts consider the classical behavior of light, including abnormal reflection and transmission
In this review, we highlight recent progress in the field of quantum optics based on metasurfaces. The review is organized as follows: we start by introducing the quantum optical effects based on surface plasmon polaritons (SPPs), we then review quantum light source based on the metasurface, manipulation of quantum state based on metasurfaces, quantum applications based on metasurfaces, and quantum vacuum engineering of quantum emitter through metasurfaces, finally we briefly discuss the future outlook of quantum optics with metasurfaces. For readers who want to know more about this topic, some other relevant reviews are recommended
Quantum plasmonics
As everyone knows, bosons and fermions are the only two types of fundamental particle in nature, they obey different statistics, and display distinguishable quantum behavior, such as coalescence or anti-coalescence for bosons and fermions respectively. For instance, photons are bosons and electrons are fermions. Something interesting happens when light is interacting with metallic nanostructure, photons couple with the free electrons on the surface of metal to generate surface plasmon polaritons (SPPs), which can be regarded as boson-like particles. So SPPs can not only provide huge local field enhancement but also inherit the quantum properties of photon rather than electron
Quantum sources
Quantum entanglement and quantum interference are the two key concepts of quantum physics that differ from classical physics. They are indeed the driving force behind quantum technologies, such as quantum communication, quantum computation, quantum metrology and quantum sensing. To employ the unique physical phenomenon for advancing the development of quantum photonic technologies, the way to acquire large-area, stable, controllable and small-scale multiphoton high dimensional quantum source is highly desirable. On the one hand, increasing the dimension of quantum source can reveal more novel quantum physics embedded in nature. On the other hand, high dimension guarantees bigger information capacity and stronger resilience to environment noise in quantum communication regime. Moreover, compared with low dimensional quantum source, high dimensional quantum source offers higher efficiency and flexibility in quantum computation.
In recent years, researchers have demonstrated high dimensional quantum source using various degrees of freedom, such as spatial mode, energy-time, polarization, path and so on
Dependent on material selection, geometry control and rotation associated with meta-atoms, optical metamaterial provides abundant freedoms to manipulate the spontaneous parametric down conversion, having potential applicability for preparing desired multiphoton quantum state. According to this, the most universal method to produce entangled photon pair at will is intrinsically available in metamaterials
Figure 1.
The density matrix reconstruction of four bell states is shown in Fig. 1(c) with high average fidelity of 97.9%. This implies that the rich phase control associated with metasurface can be fully exploited to prepare desired quantum states. Morever, because the photon pair generated from metasurface is path entangled, this complex structure can easily realize compact, stable high dimensional multiphoton quantum source. As shown in Fig. 1(d), the pump power dependencies of four-photon and six-photon source coincidence counts satisfy quadratic and cubic relationship respectively, which adequately confirms the preparation of four-photon and six-photon quantum source. Specifically, for two photon pairs independently generated from two different metalenses, one photon from each photon pair is used as the trigger, and another from each photon pair interfere at a 50∶50 fiber beam splitter, resulting in 86.3% HOM dip visibility. Optical metasurface indeed has the capability to provide a stable, compact high dimensional multiphoton quantum source. However, preparing desirable quantum states through other degree of freedoms based on optical metasurface has not been displayed and may be hopefully achieved.
Manipulation of quantum states
In the quantum photonics regime, the manipulation of quantum states is indispensable to realizing quantum logic gates. Usually, this aim can be fulfilled by linear optical elements, such as wave plates, splitters and so on. As a consequence of extraordinary ability to control phase and amplitude of light, metasurface can realize almost all functionalities provided by linear optical elements in a smaller scale and multifunctional way, so the functionality associated with linear optical devices can be directly transplanted into optical metasurface with high integration density and efficiency.
Optical metasurface has two kinds of methods to manipulate the phase of light: resonant phase and geometrical phase. For the latter, the vortex beam can be generated through designing metasurface with azimuthal phase distribution. In the quantum physics regime, this space-variant spin-dependent phase changes orbital angular momentum of photons based on the corresponding spin angular momentum, and the difference of orbital angular momentum depends on the phase distribution of metasurface. As shown in Fig. 2(a), by utilizing the geometrical phase of metasurface, photon with different polarization acquires different orbital angular momentum, the process of photons through the metasurface is presented as
Figure 2.
where
Subsequently, the entanglement between spin angular momentum and orbital angular momentum of single photon is realized. Designing metasurface with different phase distribution or flipping the metasurfacec can carry out different quantum operation on single photon. Figure 2(b) shows the four bell states of single photon with entanglement between spin angular momentum and orbital angular momentum. Based on the same principle, the entanglement of spin angular momentum of one photon with orbital angular momentum of another photon from the same photon pair is acquired, as shown in Fig. 2(c). When the spin polarization state of one photon is
Moreover, owing to the extraordinary ability of beam splitting, optical metasurfaces can also be employed to carry out projective measurements on multiphoton quantum state
Compared with all dielectric metasurface, although metallic metasurface brings inevitable loss that can reduce the coherence of light, this weakness can be transformed into advantages for particular application scenarios. For example, by designing metallic metasurface whose absorption is dependent on the polarization, the probability of specific polarization constitution of non-maximal entangled state can be reduced, which then increases the entanglement of quantum state and realizes the distillation of quantum state
Quantum optical applications
Before introducing applications of quantum optics based on metasurfaces, one important thing should be recalled is that the behavior of any type of light definitely can be described by quantum mechanics, but some quantum source can only be explained by quantum mechanics instead of classic electrodynamics, such as squeezed state, single or two photon state and so on. When the intensity of light is down to a few photons, probabilistic behavior of photon must be taken into consideration, and then many unapproachable applications in classical regime is able to be realized in quantum regime. In the classical photonic domain, the absorption of photons is probabilistic and controlled by the extinction spectrum of nanostructures. However, in the quantum regime, classical limit of absorption can be broken through combining the loss of metallic metasurfaces and quantum interference
Figure 3.
Metasurface can also be employed in quantum imaging
In the meantime, there are many application scenarios for optical metasurfaces in quantum measurement
which is a two photon NOON state. After the photon pair passes through metasurface, the left, right handed polarization photon irradiates into different direction as a consequence of geometrical phase of metasurface. Then photon pair is spatially entangled and disentangled dependent on the times of photon pair passing through metasurface. Based on this principle, P. Georgi et al. constructed an interferometer consisting of metasurface and other optical elements
Quantum vacuum engineering of quantum emitter
Initially, the lifetime of quantum emitter was thought to be intrinsic and independent of the external electromagnetic field environment until Purcell proposed that the lifetime of quantum emitter can be manipulated, e.g. the radiative rate and non-radiative rate of quantum emitters can be suppressed or enhanced by changing the electromagnetic field environment surrounding the quantum emitters. Then, the researchers began to design nanoscale optical structures with small mode volume or high Q value to enhance the interaction between quantum emitters and optical structures in space and time dimension. In the weak coupling regime, the fluorescence lifetime of quantum emitter is modulated. In the strong coupling regime, the energy levels of quantum emitters and optical structures are hybridized. Rabi splitting can be observed in absorption spectrum, scattering spectrum or fluorescence spectrum.
All of these works control the radiation behavior of quantum emitter in the near field of optical structure. The precise control of the position of quantum emitter and high loss near metallic nanostructure limits their development. In order to solve these problems, it is necessary to modulate the radiation behavior of quantum emitter in the far field of optical structure. Metasurface can achieve this goal due to its excellent phase control ability. P. K. Jha et al. systematically studied the quantum vacuum engineering of quantum emitter through metasurface
Figure 4.
Furthermore, when two quantum emitters are considered, as shown in Fig. 4(d) and 4(e). The metasurface enables the electromagnetic field radiated by the electric dipole of source point to focus on the position of the target electric dipole in a directional manner with the highest efficiency being 82%, which leads to entanglement between the two quantum emitters
Moreover, when metasurface is fully anisotropic, adjusting quantization axis of quantum emitter leads to an observable difference both in temporal dynamics and spectral properties of the quantum emitters which are initially pumped into states of opposite helicities, which confirms that optical activity can originate from the quantum interference of the multiple decay channels of quantum emitter
Conclusion and outlook
In this paper, we have summarized recent works related to quantum optics based on metasuraces. We firstly introduce some researches related to quantum plasmonics. Despite of the intrinsic loss and decoherence associated with metal nanostructure, novel quantum phenomenon, such as quantum entanglement, quantum tunneling and quantum self-interference have been demonstrated. We then review quantum source based on metasurface, high dimensional multiphoton quantum source encoded in path degree of freedom is realized. Obviously it’s just not enough to generate path-entangled photon pairs, and we need to distribute quantum states over a long distance and realize practical application of information processing. Current preferred method is via the multi-core fiber, but it’s hard to stabilize the phase difference between different channels. Alternatively, mode sorter as quantum interface to convert the path entanglement to angular orbital momentum (OAM) entanglement has been shown. However, light carried with OAM still can’t propagate in free space or fiber links over a long distance, hence, it’s necessary to convert the information encoded in path or OAM into other degrees of freedom which is more robust and stable against the environment noise. We then discuss recent techniques about manipulation of quantum states based on metasurfaces. By judiciously designing metasurfaces, the functionality of beam splitting has been utilized to enhance the speed and stability of quantum state tomography, but how to design metasurfaces to combine multiple incoherent output beams into one is still challenging and illusive. Achieving this goal can circumvent the complexities of high dimensional quantum state tomography. Moreover, we have shown that metasuface can also provide unique opportunities for quantum imaging, quantum sensing, quantum measurement and some other applications. Interestingly, although the intrinsic loss of metallic metasurfaces is detrimental to the coherence of photons, this weakness can be utilized to enhance and control the absorption of photon. Finally, we review related works about the interaction between metasurfaces and quantum emitters. The radiation behavior can be manipulated by changing the quantum vacuum surrounding the quantum emitter. Nowadays single photon source is mostly based on quantum dots as a consequence of its deterministic generation. But it still remains unknown whether single photon pair can be highly efficiently generated through the combination of metasurface and quantum emitter.
Compared with other linear optical elements, metasuface possesses richer ability to control the phase of light and smaller scale which render metasurface suitable for highly integrated quantum optical systems. The method to directly map the abundant light field manipulation functionality in the classical domain to quantum domain through metasurface is a general way to find optical metasurface’s position in quantum optics. Despite the great advances that has been achieved, the potential of metasurfaces for quantum optics has not yet been fully exploited, and some problems and challenges remain to be solved. For example, the phase encoding potential embedded in metasurface may be applied to super dense coding and quantum communication. As for manipulation of quantum states, preparing desired quantum states through electric control of metasurface is appealing and has great influence on the development of quantum communication and information. Apart from this, the most important is to know unknown, and it may be an arduous task to discover new physics through traditional optical elements. Optical metasurfaces can make experiment and measurement simple and convenient, providing a brand new and very promising platform to study quantum optics.
References
[1] VM Shalaev. Optical negative-index metamaterials. Nat Photonics, 1, 41-48(2007).
[2] J Valentine, S Zhang, T Zentgraf, E Ulin-Avila, DA Genov et al. Three-dimensional optical metamaterial with a negative refractive index. Nature, 455, 376-379(2008).
[3] P Moitra, YM Yang, Z Anderson, II Kravchenko, DP Briggs et al. Realization of an all-dielectric zero-index optical metamaterial. Nat Photonics, 7, 791-795(2013).
[4] Proceedings of the 2nd IEEE Conference on Nanotechnology 225–228 (IEEE, 2002); http://doi.org/10.1109/NANO.2002.1032233
[5] Z Jacob, LV Alekseyev, E Narimanov.
[6] ZW Liu, H Lee, Y Xiong, C Sun, X Zhang. Far-field optical hyperlens magnifying sub-diffraction-limited objects. Science, 315, 1686(2007).
[7] WS Cai, UK Chettiar, AV Kildishev, VM Shalaev. Optical cloaking with metamaterials. Nat Photonics, 1, 224-227(2007).
[8] II Smolyaninov, VN Smolyaninova, AV Kildishev, VM Shalaev. Anisotropic metamaterials emulated by tapered waveguides: application to optical cloaking. Phys Rev Lett, 102, 213901(2009).
[9] NF Yu, P Genevet, MA Kats, F Aieta, JP Tetienne et al. Light propagation with phase discontinuities: generalized laws of reflection and refraction. Science, 334, 333-337(2011).
[10] MW Klein, C Enkrich, M Wegener, S Linden. Second-harmonic generation from magnetic metamaterials. Science, 313, 502-504(2006).
[11] S Kim, J Jin, YJ Kim, IY Park, Y Kim et al. High-harmonic generation by resonant plasmon field enhancement. Nature, 453, 757-760(2008).
[12] J Lee, M Tymchenko, C Argyropoulos, PY Chen, F Lu et al. Giant nonlinear response from plasmonic metasurfaces coupled to intersubband transitions. Nature, 511, 65-69(2014).
[13] TT Luu, M Garg, SY Kruchinin, A Moulet, MT Hassan et al. Extreme ultraviolet high-harmonic spectroscopy of solids. Nature, 521, 498-502(2015).
[14] AI Kuznetsov, AE Miroshnichenko, ML Brongersma, YS Kivshar, B Luk'yanchuk. Optically resonant dielectric nanostructures. Science, 354, aag2472(2016).
[15] M Sivis, M Taucer, G Vampa, K Johnston, A Staudte et al. Tailored semiconductors for high-harmonic optoelectronics. Science, 357, 303-306(2017).
[16] K Koshelev, S Kruk, E Melik-Gaykazyan, JH Choi, A Bogdanov et al. Subwavelength dielectric resonators for nonlinear nanophotonics. Science, 367, 288-292(2020).
[17] ZE Bomzon, G Biener, V Kleiner, E Hasman. Space-variant Pancharatnam–Berry phase optical elements with computer-generated subwavelength gratings. Opt Lett, 27, 1141-1143(2002).
[18] AV Zayats, II Smolyaninov, AA Maradudin. Nano-optics of surface plasmon polaritons. Phys Rep, 408, 131-314(2005).
[19] A Bouhelier, M Beversluis, A Hartschuh, L Novotny. Near-field second-harmonic generation induced by local field enhancement. Phys Rev Lett, 90, 013903(2003).
[20] EM Kim, SS Elovikov, TV Murzina, AA Nikulin, OA Aktsipetrov et al. Surface-enhanced optical third-harmonic generation in Ag island films. Phys Rev Lett, 95, 227402(2005).
[21] A Kinkhabwala, ZF Yu, SH Fan, Y Avlasevich, K Müllen et al. Large single-molecule fluorescence enhancements produced by a bowtie nanoantenna. Nat Photonics, 3, 654-657(2009).
[22] J Renger, R Quidant, Hulst van, L Novotny. Surface-enhanced nonlinear four-wave mixing. Phys Rev Lett, 104, 046803(2010).
[23] IY Park, S Kim, J Choi, DH Lee, YJ Kim et al. Plasmonic generation of ultrashort extreme-ultraviolet light pulses. Nat Photonics, 5, 677-681(2011).
[24] M Sivis, M Duwe, B Abel, C Ropers. Extreme-ultraviolet light generation in plasmonic nanostructures. Nat Phys, 9, 304-309(2013).
[25] H Aouani, M Rahmani, M Navarro-Cia, SA Maier. Third-harmonic-upconversion enhancement from a single semiconductor nanoparticle coupled to a plasmonic antenna. Nat Nanotechnol, 9, 290-294(2014).
[26] WQ Zhu, KB Crozier. Quantum mechanical limit to plasmonic enhancement as observed by surface-enhanced Raman scattering. Nat Commun, 5, 5228(2014).
[27] S Han, H Kim, YW Kim, YJ Kim, S Kim et al. High-harmonic generation by field enhanced femtosecond pulses in metal-sapphire nanostructure. Nat Commun, 7, 13105(2016).
[28] JH Zhong, J Vogelsang, JM Yi, D Wang, L Wittenbecher et al. Nonlinear plasmon-exciton coupling enhances sum-frequency generation from a hybrid metal/semiconductor nanostructure. Nat Commun, 11, 1464(2020).
[29] XY Zhang, QT Cao, Z Wang, YX Liu, CW Qiu et al. Symmetry-breaking-induced nonlinear optics at a microcavity surface. Nat Photonics, 13, 21-24(2019).
[30] AE Miroshnichenko, AB Evlyukhin, YF Yu, RM Bakker, A Chipouline et al. Nonradiating anapole modes in dielectric nanoparticles. Nat Commun, 6, 8069(2015).
[31] G Grinblat, Y Li, MP Nielsen, RF Oulton, SA Maier. Enhanced third harmonic generation in single germanium nanodisks excited at the anapole mode. Nano Lett, 16, 4635-4640(2016).
[32] L Carletti, K Koshelev, Angelis De, Y Kivshar. Giant nonlinear response at the nanoscale driven by bound states in the continuum. Phys Rev Lett, 121, 033903(2018).
[33] ZJ Liu, Y Xu, Y Lin, J Xiang, TH Feng et al. High-Q quasibound states in the continuum for nonlinear metasurfaces. Phys Rev Lett, 123, 253901(2019).
[34] YM Yang, WY Wang, A Boulesbaa, II Kravchenko, DP Briggs et al. Nonlinear fano-resonant dielectric metasurfaces. Nano Lett, 15, 7388-7393(2015).
[35] L Carletti, A Locatelli, O Stepanenko, G Leo, Angelis De. Enhanced second-harmonic generation from magnetic resonance in AlGaAs nanoantennas. Opt Express, 23, 26544-26550(2015).
[36] MR Shcherbakov, DN Neshev, B Hopkins, AS Shorokhov, I Staude et al. Enhanced third-harmonic generation in silicon nanoparticles driven by magnetic response. Nano Lett, 14, 6488-6492(2014).
[37] S Kruk, A Poddubny, D Smirnova, L Wang, A Slobozhanyuk et al. Nonlinear light generation in topological nanostructures. Nat Nanotechnol, 14, 126-130(2019).
[38] D Smirnova, S Kruk, D Leykam, E Melik-Gaykazyan, DY Choi et al. Third-harmonic generation in photonic topological metasurfaces. Phys Rev Lett, 123, 103901(2019).
[39] SM Wang, PC Wu, VC Su, YC Lai, CH Chu et al. Broadband achromatic optical metasurface devices. Nat Commun, 8, 187(2017).
[40] SM Wang, PC Wu, VC Su, YC Lai, MK Chen et al. A broadband achromatic metalens in the visible. Nat Nanotechnol, 13, 227-232(2018).
[41] WM Ye, F Zeuner, X Li, B Reineke, S He et al. Spin and wavelength multiplexed nonlinear metasurface holography. Nat Commun, 7, 11930(2016).
[42] XJ Ni, AV Kildishev, VM Shalaev. Metasurface holograms for visible light. Nat Commun, 4, 2807(2013).
[43] GX Zheng, H Mühlenbernd, M Kenney, GX Li, T Zentgraf et al. Metasurface holograms reaching 80% efficiency. Nat Nanotechnol, 10, 308-312(2015).
[44] LL Huang, XZ Chen, H Mühlenbernd, H Zhang, SM Chen et al. Three-dimensional optical holography using a plasmonic metasurface. Nat Commun, 4, 2808(2013).
[45] XB Yin, ZL Ye, J Rho, Y Wang, X Zhang. Photonic spin Hall effect at metasurfaces. Science, 339, 1405-1407(2013).
[46] YM Yang, WY Wang, P Moitra, II Kravchenko, DP Briggs et al. Dielectric meta-reflectarray for broadband linear polarization conversion and optical vortex generation. Nano Lett, 14, 1394-1399(2014).
[47] E Karimi, SA Schulz, Leon De, H Qassim, J Upham et al. Generating optical orbital angular momentum at visible wavelengths using a plasmonic metasurface. Light Sci Appl, 3, e167(2014).
[48] NJ Cerf, M Bourennane, A Karlsson, N Gisin. Security of quantum key distribution using d-level systems. Phys Rev Lett, 88, 127902(2002).
[49] N Gisin, GG Ribordy, W Tittel, H Zbinden. Quantum cryptography. Rev Mod Phys, 74, 145-195(2002).
[50] N Gisin, R Thew. Quantum communication. Nat Photonics, 1, 165-171(2007).
[51] V Scarani, H Bechmann-Pasquinucci, NJ Cerf, M Dušek, N Lütkenhaus et al. The security of practical quantum key distribution. Rev Mod Phys, 81, 1301-1350(2009).
[52] HK Lo, M Curty, K Tamaki. Secure quantum key distribution. Nat Photonics, 8, 595-604(2014).
[53] XL Wang, XD Cai, ZE Su, MC Chen, D Wu et al. Quantum teleportation of multiple degrees of freedom of a single photon. Nature, 518, 516-519(2015).
[54] E Knill, R Laflamme, GJ Milburn. A scheme for efficient quantum computation with linear optics. Nature, 409, 46-52(2001).
[55] JL O'Brien. Optical quantum computing. Science, 318, 1567-1570(2007).
[56] BP Lanyon, M Barbieri, MP Almeida, T Jennewein, TC Ralph et al. Simplifying quantum logic using higher-dimensional Hilbert spaces. Nat Phys, 5, 134-140(2009).
[57] M Neeley, M Ansmann, RC Bialczak, M Hofheinz, E Lucero et al. Emulation of a quantum spin with a superconducting phase qudit. Science, 325, 722-725(2009).
[58] R Kaltenbaek, J Lavoie, B Zeng, SD Bartlett, KJ Resch. Optical one-way quantum computing with a simulated valence-bond solid. Nat Phys, 6, 850-854(2010).
[59] A Aspuru-Guzik, P Walther. Photonic quantum simulators. Nat Phys, 8, 285-291(2012).
[60] IM Georgescu, S Ashhab, F Nori. Quantum simulation. Rev Mod Phys, 86, 153-185(2014).
[61] V Giovannetti, S Lloyd, L Maccone. Advances in quantum metrology. Nat Photonics, 5, 222-229(2011).
[62] S Pirandola, BR Bardhan, T Gehring, C Weedbrook, S Lloyd. Advances in photonic quantum sensing. Nat Photonics, 12, 724-733(2018).
[63] JQ You, F Nori. Atomic physics and quantum optics using superconducting circuits. Nature, 474, 589-597(2011).
[64] S Diehl, A Micheli, A Kantian, B Kraus, H Büchler et al. Quantum states and phases in driven open quantum systems with cold atoms. Nat Phys, 4, 878-883(2008).
[65] D Leibfried, R Blatt, C Monroe, D Wineland. Quantum dynamics of single trapped ions. Rev Mod Phys, 75, 281-324(2003).
[66] CL Li, P Yu, YJ Huang, Q Zhou, J Wu et al. Dielectric metasurfaces: from wavefront shaping to quantum platforms. Prog Surf Sci, 95, 100584(2020).
[67] arXiv: 2006.03757 (2020).
[68] N Rivera, I Kaminer. Light-matter interactions with photonic quasiparticles. Nat Rev Phys, 2, 538-561(2020).
[69] arXiv: 2007.14722 (2020).
[70] RW Heeres, LP Kouwenhoven, V Zwiller. Quantum interference in plasmonic circuits. Nat Nanotechnol, 8, 719-722(2013).
[71] JS Fakonas, H Lee, YA Kelaita, HA Atwater. Two-plasmon quantum interference. Nat Photonics, 8, 317-320(2014).
[72] E Altewischer, Exter van, JP Woerdman. Plasmon-assisted transmission of entangled photons. Nature, 418, 304-306(2002).
[73] E Moreno, FJ García-Vidal, D Erni, JI Cirac, L Martín-Moreno. Theory of plasmon-assisted transmission of entangled photons. Phys Rev Lett, 92, 236801(2004).
[74] S Fasel, F Robin, E Moreno, D Erni, N Gisin et al. Energy-time entanglement preservation in plasmon-assisted light transmission. Phys Rev Lett, 94, 110501(2005).
[75] A Huck, S Smolka, P Lodahl, AS Sørensen, A Boltasseva et al. Demonstration of quadrature-squeezed surface plasmons in a gold waveguide. Phys Rev Lett, 102, 246802(2009).
[76] SF Tan, L Wu, JKW Yang, P Bai, M Bosman et al. Quantum plasmon resonances controlled by molecular tunnel junctions. Science, 343, 1496-1499(2014).
[77] R Kolesov, B Grotz, G Balasubramanian, RJ Stöhr, AAL Nicolet et al. Wave–particle duality of single surface plasmon polaritons. Nat Phys, 5, 470-474(2009).
[78] MC Dheur, E Devaux, TW Ebbesen, A Baron, JC Rodier et al. Single-plasmon interferences. Sci Adv, 2, e1501574(2016).
[79] M Erhard, M Krenn, A Zeilinger. Advances in high-dimensional quantum entanglement. Nat Rev Phys, 2, 365-381(2020).
[80] M Reck, A Zeilinger, HJ Bernstein, P Bertani. Experimental realization of any discrete unitary operator. Phys Rev Lett, 73, 58-61(1994).
[81] M Krenn, A Hochrainer, M Lahiri, A Zeilinger. Entanglement by path identity. Phys Rev Lett, 118, 080401(2017).
[82] JW Wang, S Paesani, YH Ding, R Santagati, P Skrzypczyk et al. Multidimensional quantum entanglement with large-scale integrated optics. Science, 360, 285-291(2018).
[83] M Siomau, AA Kamli, SA Moiseev, BC Sanders. Entanglement creation with negative index metamaterials. Phys Rev A, 85, 050303(2012).
[84] AN Poddubny, IV Iorsh, AA Sukhorukov. Generation of photon-plasmon quantum states in nonlinear hyperbolic metamaterials. Phys Rev Lett, 117, 123901(2016).
[85] G Marino, AS Solntsev, L Xu, VF Gili, L Carletti et al. Spontaneous photon-pair generation from a dielectric nanoantenna. Optica, 6, 1416-1422(2019).
[86] arXiv: 2009.00324 (2020).
[87] Y Ming, W Zhang, J Tang, Y Liu, ZL Xia et al. Photonic entanglement based on nonlinear metamaterials. Laser Photonics Rev, 14, 1900146(2020).
[88] L Li, ZX Liu, XF Ren, SM Wang, VC Su et al. Metalens-array-based high-dimensional and multiphoton quantum source. Science, 368, 1487-1490(2020).
[89] T Stav, A Faerman, E Maguid, D Oren, V Kleiner et al. Quantum entanglement of the spin and orbital angular momentum of photons using metamaterials. Science, 361, 1101-1104(2018).
[90] K Wang, JG Titchener, SS Kruk, L Xu, HP Chung et al. Quantum metasurface for multiphoton interference and state reconstruction. Science, 361, 1104-1108(2018).
[91] M Asano, M Bechu, M Tame, Ş Özdemir, R Ikuta et al. Distillation of photon entanglement using a plasmonic metamaterial. Sci Rep, 5, 18313(2015).
[92] SA Uriri, T Tashima, X Zhang, M Asano, M Bechu et al. Active control of a plasmonic metamaterial for quantum state engineering. Phys Rev A, 97, 053810(2018).
[93] T Roger, S Vezzoli, E Bolduc, J Valente, JJF Heitz et al. Coherent perfect absorption in deeply subwavelength films in the single-photon regime. Nat Commun, 6, 7031(2015).
[94] C Altuzarra, S Vezzoli, J Valente, WB Gao, C Soci et al. Coherent perfect absorption in metamaterials with entangled photons. ACS Photonics, 4, 2124-2128(2017).
[95] A Lyons, D Oren, T Roger, V Savinov, J Valente et al. Coherent metamaterial absorption of two-photon states with 40% efficiency. Phys Rev A, 99, 011801(2019).
[96] C Altuzarra, A Lyons, GH Yuan, C Simpson, T Roger et al. Imaging of polarization-sensitive metasurfaces with quantum entanglement. Phys Rev A, 99, 020101(2019).
[97] JX Zhou, SK Liu, HL Qian, YH Li, HL Luo et al. Metasurface enabled quantum edge detection. Sci Adv, 6, eabc4385(2020).
[98] P Georgi, M Massaro, KH Luo, B Sain, N Montaut et al. Metasurface interferometry toward quantum sensors. Light Sci Appl, 8, 70(2019).
[99] SZ Chen, XX Zhou, CQ Mi, ZX Liu, HL Luo et al. Dielectric metasurfaces for quantum weak measurements. Appl Phys Lett, 110, 161115(2017).
[100] JS Lundeen, B Sutherland, A Patel, C Stewart, C Bamber. Direct measurement of the quantum wavefunction. Nature, 474, 188-191(2011).
[101] O Hosten, P Kwiat. Observation of the spin hall effect of light via weak measurements. Science, 319, 787-790(2008).
[102] PK Jha, XJ Ni, C Wu, Y Wang, X Zhang. Metasurface-enabled remote quantum interference. Phys Rev Lett, 115, 025501(2015).
[103] PK Jha, N Shitrit, J Kim, XX Ren, Y Wang et al. Metasurface-mediated quantum entanglement. ACS Photonics, 5, 971-976(2018).
[104] PK Jha, N Shitrit, XX Ren, Y Wang, X Zhang. Spontaneous exciton valley coherence in transition metal dichalcogenide monolayers interfaced with an anisotropic metasurface. Phys Rev Lett, 121, 116102(2018).
[105] D Kornovan, M Petrov, I Iorsh. Noninverse dynamics of a quantum emitter coupled to a fully anisotropic environment. Phys Rev A, 100, 033840(2019).
[106] E Lassalle, P Lalanne, S Aljunid, P Genevet, B Stout et al. Long-lifetime coherence in a quantum emitter induced by a metasurface. Phys Rev A, 101, 013837(2020).
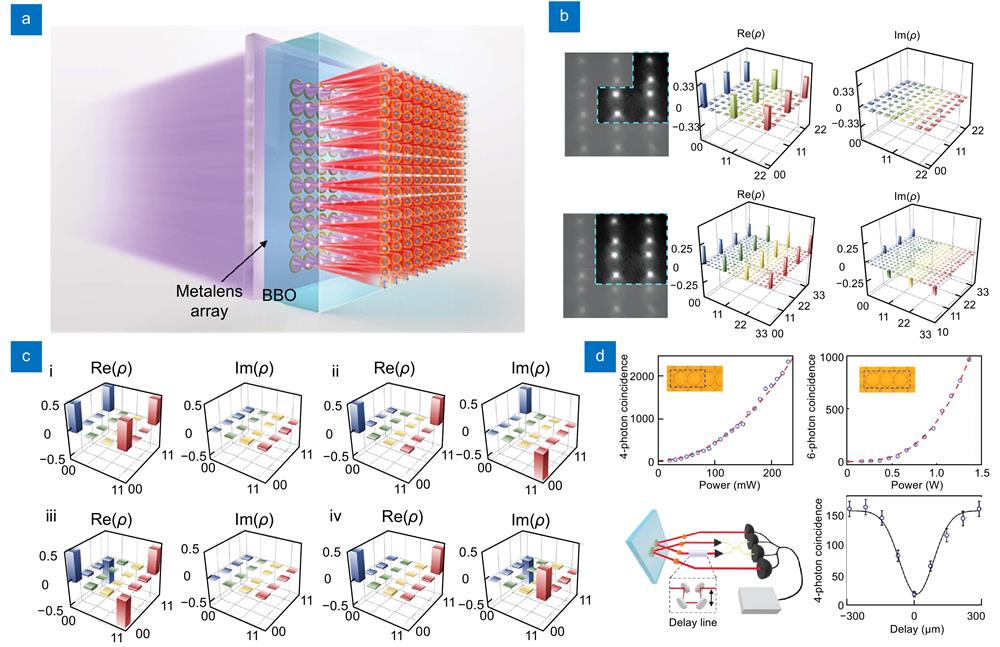
Set citation alerts for the article
Please enter your email address