
- Photonics Research
- Vol. 10, Issue 7, 1731 (2022)
Abstract
1. INTRODUCTION
Metasurfaces, as 2D metamaterials consisting of an array of function-driven artificial meta-atoms, have attracted tremendous attention due to their unprecedented abilities in manipulating the phase, polarization, and amplitude of electromagnetic (EM) waves [1]. In the terahertz (THz) frequency band, metasurfaces have resulted in numerous applications, including absorbers [2], metalenses [3], beam steering [4], holography [5,6], vector beams [7], topological waveguides [8], bound states in the continuum [9,10], etc. Despite metasurfaces having exhibited clear advantages over classical optical components, their performances are fixed upon fabrication, which limits their further applications. So actively tuning the properties of EM waves is highly desirable for integrated photonic systems [11,12]. Currently, various active modulation methods have been proposed, such as liquid crystals (LCs) [13–15], semiconductors [16–18], microelectromechanical system (MEMS) [19–21], graphene [22–24], and phase-change material (PCM) [25–29]. These mechanisms of active modulation have led to a diverse range of tunable THz metasurfaces [13,16,17,20,22–24,26–29]. However, a constant stimulus is needed to preserve the working states of devices, and there has yet to be developed an energy-efficient solution for maintaining the desired function with zero static power consumption in the THz regime. Moreover, most of these approaches are limited to a single functionality, which hinders their further applications.
In this work, we demonstrate a novel class of nonvolatile, reconfigurable, and dynamic Janus metasurfaces by incorporating PCM GST in the THz regime, which can offer a wealth of switchable functionalities. The metasurfaces comprise three sets of functional structures arranged using multiplex methods: two active sets composed of GST integrated C-shaped slit resonators (G-CRs) and a passive set composed of CRs. In the amorphous state of GST, the function of the device depends on the contribution of three sets of structures; however, the function is governed by the passive set in the crystalline state. Here, we experimentally show the reversible switching characteristic of GST on large areas by applying a single ns-laser pulse with a diameter of 1 cm to achieve amorphization of GST and heating the entire sample on a hot plate to induce crystallization. Based on this scheme, several important bifunctional metasurfaces are designed, fabricated, and experimentally characterized, including dynamic beam splitter, bifocal metalens, dual-mode focusing optical vortex (FOV) generators, and switchable metalens/FOV generators. Experimental results show that the proposed metasurfaces can realize two independent functionalities in both states and can be switched reversibly and repeatedly. Moreover, the function in a certain phase is stable with zero hold power at room temperature. The proposed nonvolatile, reconfigurable, and dynamic Janus metasurfaces combined with GST provide a novel way to realize multifunctional and compact THz devices and may have essential applications in THz integrated devices, imaging, and communications.
Sign up for Photonics Research TOC. Get the latest issue of Photonics Research delivered right to you!Sign up now
2. RESULTS
A. Design Principles
A schematic diagram of the proposed nonvolatile, reconfigurable Janus metasurface is shown in Fig. 1(a), which demonstrates the reversible switching between the
Figure 1.Operation principle of dynamic Janus metasurface. (a) Schematic demonstration of a nonvolatile reconfigurable Janus metasurface consisting of three sets of structures,
The working principle is presented in Fig. 1(a). The metasurface consists of three sets of functional structures arranged using multiplex methods, as shown in the black rectangular box: two active sets (
B. Nonvolatile Dynamic Reversible Switching of Bifunctional Metasurfaces
First, a dynamic beam splitter is demonstrated using our Janus metasurface, as shown in Fig. 2(a). The anomalous refraction of cross-polarization THz waves propagates along two opposite directions in crystalline and amorphous states of GST at normal incidence of
Figure 2.Dynamic beam steering. (a) Schematic principle of the proposed dynamic beam splitter, which can steer cross-polarization THz waves toward two opposite directions in crystalline and amorphous states. (b) Optical microscope image of part of the fabricated metasurface and enlarged views of basic elements. The sample is composed of three sets of structures:
Second, a dynamic bifocal metalens is proposed that exhibits switchable focal lengths in amorphous and crystalline states of GST, as depicted in Fig. 3(a). Structure sets
Figure 3.Dynamic bifocal metalens. (a) Schematic principle of the proposed dynamic bifocal metalens, which exhibits switchable focal lengths in amorphous and crystalline states of GST. (b) Optical microscope image of part of the fabricated metasurface and enlarged views of basic elements. The sample is composed of three sets of structures:
Next, an FOV generator with dual-mode functionality is proposed, which exhibits switchable FOV modes in amorphous and crystalline states of GST, as illustrated in Fig. 4(a). As mentioned above, the metasurface is composed of three sets of structures, in which
Figure 4.Dual-mode FOV generator. (a) Schematic principle of dual-mode FOV generator, which exhibits different values of topological charge in amorphous and crystalline states of GST. (b) Optical microscope image of part of the fabricated metasurface and enlarged views of basic elements. The radius of the fabricated metasurface is 5120 μm. Measured normalized intensity distribution of (c)–(e)
The above devices are all switching between the same functions. To verify the versatility of the designed device, as a proof of concept, beam switching between different functionalities depending on the state of GST is proposed, as illustrated in Fig. 5(a). The metasurface acts as an FOV generator in the amorphous state and as a metalens in the crystalline state. Structures
Figure 5.Dual-functionality metasurface. (a) Schematic principle of dual-functionality metasurface, which functions as an FOV generator in the amorphous state and as a metalens in the crystalline state of GST. (b) Optical microscope image of part of the fabricated metasurface and enlarged views of basic elements. The radius of the fabricated metasurface is 5120 μm. (c)–(e) Measured normalized intensity distributions of
3. METHODS
A. Sample Fabrication
High-resistivity Si wafers with a thickness of 2 mm were used as substrates. Before deposition, the substrates were cleaned ultrasonically in acetone, isopropanol, and deionized water in sequence. A 100-nm-thick GST film was first sputter-deposited on the Si substrate. Conventional photolithography was used to attain desired GST patterns. The second photolithography was carried out and a 200-nm-thick Al was subsequently deposited on top of the GST film. Finally, the lift-off procedure was adopted to attain desired CR patterns.
B. Experimental Characterization
The dynamic beam splitter is characterized using FAT-TDS. The THz waves are transmitted and detected by a pair of commercially available photoconductive antennas. The generated THz radiation is collimated and normally illuminated on the sample, which is fixed at the center of a rotation stage. Additionally, three metallic-grid-based THz linear polarizers are employed to attain the desired polarization. Two are placed before the sample and used to allow 45°-polarized and
The bifocal metalens, dual-mode FOV generators, and switchable metalens/FOV generators are characterized by an NSTM system. The THz waves are generated by a homemade photoconductive antenna and detected by a commercially available THz near-field probe. To enable a 3D scan of the THz electric field, the probe is mounted on a 2D translation stage, and the sample is on a 3D translation stage. Same as the FAT-TDS system, three polarizers are used to allow only
4. CONCLUSION
In conclusion, we have proposed dynamic Janus metasurfaces based on a multiplex scheme comprising two sets of active structures and a passive set. With the help of optical and thermal stimuli, G-CRs can be reversibly switched between amorphous and crystalline states, which contributes to switching between different functions. As a proof of concept, dynamic beam splitter, bifocal metalens, dual-mode FOV generator, and switchable beam between an FOV beam and a converging beam are successfully designed, fabricated, and experimentally characterized. Our devices exhibit great reconfigurability, which features repeatable switching between two different functions, and nonvolatility without constant stimuli to maintain compared with other PCMs (e.g.,
Acknowledgment
Acknowledgment. Z. Tian conceived the idea. S. Zhang conducted the design. H. Li and K. Liu deposited the GST films. Y. Xu and Q. Wang helped with the sample preparation. S. Zhang, X. Chen, Y. Xu, and X. Jiang performed the measurements. S. Zhang wrote the manuscript. Z. Tian and T. Cao revised the manuscript and supervised the project. All authors discussed the results and commented on the paper.
APPENDIX A: TRANSMISSION AND CONDUCTIVITY OF GST DURING MULTIPLE SWITCHING CYCLES
We experimentally characterized the THz transmission of GST film in amorphous and crystalline states. A 100-nm-thick GST thin film is deposited on a 2-mm-thick high-resistivity Si substrate. Here, the crystalline-to-amorphous transition of GST is induced by a single
Figure 6.(a) Transmission at 0.8 THz of the same GST sample during multiple switching cycles. Inset: atom distribution diagrams of the two phases of GST. (b) Calculated conductivities in amorphous and crystalline states of GST.
APPENDIX B: SIMULATED AMPLITUDE AND PHASE SHIFT OF BASIC CELLS
Figure
Figure 7.(a) Simulated amplitude and phase shift of eight G-CRs in the amorphous state and amplitude in the crystalline state of GST at 0.8 THz. (b) Simulated amplitude and phase shift of eight CRs. The orientation angles of the former four CRs,
APPENDIX C: DUAL-MODE FOV GENERATOR WITH DIFFERENT VALUES OF TOPOLOGICAL CHARGE
An FOV generator with modes of
Figure 8.(a), (h) Optical microscope image of part of the fabricated metasurface and enlarged views of basic elements. The radius of the fabricated metasurface is 5120 μm. (b)–(d), (i)–(k) Measured normalized intensity distributions of
APPENDIX D: SWITCHING PERFORMANCE OF THE DEVICE
To demonstrate the splitting ratios of the beam splitter in both states of GST, the splitting ratios are calculated by the following equation:
Figure 9.Broadband splitting ratios in both states of GST.
Figure 10.Calculated purity of different vortex beams.
We also calculate and extract the purity of generated vortex beams and normalized intensity profiles of the horizontal and vertical cross sections from Fig.
References
[1] C.-W. Qiu, T. Zhang, G. Hu, Y. Kivshar. Quo vadis, metasurfaces?. Nano Lett., 21, 5461-5474(2021).
[2] X. Chen, Z. Tian, Y. Lu, Y. Xu, X. Zhang, C. Ouyang, J. Gu, J. Han, W. Zhang. Electrically tunable perfect terahertz absorber based on a graphene Salisbury screen hybrid metasurface. Adv. Opt. Mater., 8, 1900660(2020).
[3] F. Zhao, Z. Li, X. Dai, X. Liao, S. Li, J. Cao, Z. Shang, Z. Zhang, G. Liang, G. Chen. Broadband achromatic sub-diffraction focusing by an amplitude-modulated terahertz metalens. Adv. Opt. Mater., 8, 2000842(2020).
[4] L. Cong, Y. K. Srivastava, H. Zhang, X. Zhang, J. Han, R. Singh. All-optical active THz metasurfaces for ultrafast polarization switching and dynamic beam splitting. Light Sci. Appl., 7, 28(2018).
[5] S. Venkatesh, X. Lu, H. Saeidi, K. Sengupta. A high-speed programmable and scalable terahertz holographic metasurface based on tiled CMOS chips. Nat. Electron., 3, 785-793(2020).
[6] Q. Wang, E. Plum, Q. Yang, X. Zhang, Q. Xu, Y. Xu, J. Han, W. Zhang. Reflective chiral meta-holography: multiplexing holograms for circularly polarized waves. Light Sci. Appl., 7, 25(2018).
[7] Y. Xu, H. Zhang, Q. Li, X. Zhang, Q. Xu, W. Zhang, C. Hu, X. Zhang, J. Han, W. Zhang. Generation of terahertz vector beams using dielectric metasurfaces via spin-decoupled phase control. Nanophotonics, 9, 3393-3402(2020).
[8] Y. Yang, Y. Yamagami, X. Yu, P. Pitchappa, J. Webber, B. Zhang, M. Fujita, T. Nagatsuma, R. Singh. Terahertz topological photonics for on-chip communication. Nat. Photonics, 14, 446-451(2020).
[9] X. Zhao, C. Chen, K. Kaj, I. Hammock, Y. Huang, R. D. Averitt, X. Zhang. Terahertz investigation of bound states in the continuum of metallic metasurfaces. Optica, 7, 1548-1554(2020).
[10] S. Han, L. Cong, Y. K. Srivastava, B. Qiang, M. V. Rybin, A. Kumar, R. Jain, W. X. Lim, V. G. Achanta, S. S. Prabhu. All-dielectric active terahertz photonics driven by bound states in the continuum. Adv. Mater., 31, 1901921(2019).
[11] A. Nemati, Q. Wang, M. Hong, J. Teng. Tunable and reconfigurable metasurfaces and metadevices. Opto-Electron. Adv., 1, 18000901(2018).
[12] Z. Gong, F. Yang, L. Wang, R. Chen, J. Wu, C. P. Grigoropoulos, J. Yao. Phase change materials in photonic devices. J. Appl. Phys., 129, 030902(2021).
[13] L. Wang, X.-W. Lin, W. Hu, G.-H. Shao, P. Chen, L.-J. Liang, B.-B. Jin, P.-H. Wu, H. Qian, Y.-N. Lu. Broadband tunable liquid crystal terahertz waveplates driven with porous graphene electrodes. Light Sci. Appl., 4, e253(2015).
[14] F. Hu, Y. Deng, W. Saad, M. Bennis, A. H. Aghvami. Cellular-connected wireless virtual reality: requirements, challenges, and solutions. IEEE Commun. Mag., 58, 105-111(2020).
[15] I. Kim, M. A. Ansari, M. Q. Mehmood, W. S. Kim, J. Jang, M. Zubair, Y. K. Kim, J. Rho. Stimuli-responsive dynamic metaholographic displays with designer liquid crystal modulators. Adv. Mater., 32, 2004664(2020).
[16] H.-T. Chen, W. J. Padilla, J. M. Zide, A. C. Gossard, A. J. Taylor, R. D. Averitt. Active terahertz metamaterial devices. Nature, 444, 597-600(2006).
[17] J. Zhou, D. R. Chowdhury, R. Zhao, A. K. Azad, H.-T. Chen, C. M. Soukoulis, A. J. Taylor, J. F. O’Hara. Terahertz chiral metamaterials with giant and dynamically tunable optical activity. Phys. Rev. B, 86, 035448(2012).
[18] L. Wang, M. Eliceiri, Y. Deng, Y. Rho, W. Shou, H. Pan, J. Yao, C. P. Grigoropoulos. Fast reversible phase change silicon for visible active photonics. Adv. Funct. Mater., 30, 1910784(2020).
[19] E. Arbabi, A. Arbabi, S. M. Kamali, Y. Horie, M. Faraji-Dana, A. Faraon. MEMS-tunable dielectric metasurface lens. Nat. Commun., 9, 812(2018).
[20] M. Manjappa, P. Pitchappa, N. Singh, N. Wang, N. I. Zheludev, C. Lee, R. Singh. Reconfigurable MEMS Fano metasurfaces with multiple-input–output states for logic operations at terahertz frequencies. Nat. Commun., 9, 4056(2018).
[21] C. Meng, P. C. Thrane, F. Ding, J. Gjessing, M. Thomaschewski, C. Wu, C. Dirdal, S. I. Bozhevolnyi. Dynamic piezoelectric MEMS-based optical metasurfaces. Sci. Adv., 7, eabg5639(2021).
[22] S. H. Lee, M. Choi, T.-T. Kim, S. Lee, M. Liu, X. Yin, H. K. Choi, S. S. Lee, C.-G. Choi, S.-Y. Choi. Switching terahertz waves with gate-controlled active graphene metamaterials. Nat. Mater., 11, 936-941(2012).
[23] Q. Li, Z. Tian, X. Zhang, R. Singh, L. Du, J. Gu, J. Han, W. Zhang. Active graphene–silicon hybrid diode for terahertz waves. Nat. Commun., 6, 7082(2015).
[24] X. Chen, Z. Tian, J. Wang, Y. Yuan, X. Zhang, C. Ouyang, J. Gu, J. Han, W. Zhang. Hysteretic behavior in ion gel-graphene hybrid terahertz modulator. Carbon, 155, 514-520(2019).
[25] L. Liu, X. Zhang, M. Kenney, X. Su, N. Xu, C. Ouyang, Y. Shi, J. Han, W. Zhang, S. Zhang. Broadband metasurfaces with simultaneous control of phase and amplitude. Adv. Mater., 26, 5031-5036(2014).
[26] M. Liu, E. Plum, H. Li, S. Duan, S. Li, Q. Xu, X. Zhang, C. Zhang, C. Zou, B. Jin. Switchable chiral mirrors. Adv. Opt. Mater., 8, 2000247(2020).
[27] P. Pitchappa, A. Kumar, S. Prakash, H. Jani, T. Venkatesan, R. Singh. Chalcogenide phase change material for active terahertz photonics. Adv. Mater., 31, 1808157(2019).
[28] K. Makino, K. Kato, Y. Saito, P. Fons, A. V. Kolobov, J. Tominaga, T. Nakano, M. Nakajima. Terahertz spectroscopic characterization of Ge2Sb2Te5 phase change materials for photonics applications. J. Mater. Chem. C, 7, 8209-8215(2019).
[29] P. Pitchappa, A. Kumar, S. Prakash, H. Jani, R. Medwal, M. Mishra, R. S. Rawat, T. Venkatesan, N. Wang, R. Singh. Volatile ultrafast switching at multilevel nonvolatile states of phase change material for active flexible terahertz metadevices. Adv. Funct. Mater., 31, 2100200(2021).
[30] M. Wuttig, N. Yamada. Phase-change materials for rewriteable data storage. Nat. Mater., 6, 824-832(2007).
[31] A. V. Kolobov, P. Fons, A. I. Frenkel, A. L. Ankudinov, J. Tominaga, T. Uruga. Understanding the phase-change mechanism of rewritable optical media. Nat. Mater., 3, 703-708(2004).
[32] S. Abdollahramezani, O. Hemmatyar, H. Taghinejad, A. Krasnok, Y. Kiarashinejad, M. Zandehshahvar, A. Alù, A. Adibi. Tunable nanophotonics enabled by chalcogenide phase-change materials. Nanophotonics, 9, 1189-1241(2020).
[33] M. Wuttig, H. Bhaskaran, T. Taubner. Phase-change materials for non-volatile photonic applications. Nat. Photonics, 11, 465-476(2017).
[34] C. Ríos, M. Stegmaier, P. Hosseini, D. Wang, T. Scherer, C. D. Wright, H. Bhaskaran, W. H. Pernice. Integrated all-photonic non-volatile multi-level memory. Nat. Photonics, 9, 725-732(2015).
[35] Z. Cheng, C. Ríos, N. Youngblood, C. D. Wright, W. H. Pernice, H. Bhaskaran. Device-level photonic memories and logic applications using phase-change materials. Adv. Mater., 30, 1802435(2018).
[36] N. Farmakidis, N. Youngblood, X. Li, J. Tan, J. L. Swett, Z. Cheng, C. D. Wright, W. H. Pernice, H. Bhaskaran. Plasmonic nanogap enhanced phase-change devices with dual electrical-optical functionality. Sci. Adv., 5, eaaw2687(2019).
[37] T. Tuma, A. Pantazi, M. Le Gallo, A. Sebastian, E. Eleftheriou. Stochastic phase-change neurons. Nat. Nanotechnol., 11, 693-699(2016).
[38] J. Feldmann, M. Stegmaier, N. Gruhler, C. Ríos, H. Bhaskaran, C. Wright, W. Pernice. Calculating with light using a chip-scale all-optical abacus. Nat. Commun., 8, 1256(2017).
[39] Z. Cheng, C. Ríos, W. H. Pernice, C. D. Wright, H. Bhaskaran. On-chip photonic synapse. Sci. Adv., 3, e1700160(2017).
[40] P. Hosseini, C. D. Wright, H. Bhaskaran. An optoelectronic framework enabled by low-dimensional phase-change films. Nature, 511, 206-211(2014).
[41] K.-K. Du, Q. Li, Y.-B. Lyu, J.-C. Ding, Y. Lu, Z.-Y. Cheng, M. Qiu. Control over emissivity of zero-static-power thermal emitters based on phase-changing material GST. Light Sci. Appl., 6, e16194(2017).
[42] C. R. de Galarreta, I. Sinev, A. M. Alexeev, P. Trofimov, K. Ladutenko, S. G.-C. Carrillo, E. Gemo, A. Baldycheva, J. Bertolotti, C. D. Wright. Reconfigurable multilevel control of hybrid all-dielectric phase-change metasurfaces. Optica, 7, 476-484(2020).
[43] L. Mao, Y. Li, G. Li, S. Zhang, T. Cao. Reversible switching of electromagnetically induced transparency in phase change metasurfaces. Adv. Photon., 2, 056004(2020).
[44] P. Yu, J. Li, S. Zhang, Z. Jin, G. Schütz, C.-W. Qiu, M. Hirscher, N. J. Liu. Dynamic Janus metasurfaces in the visible spectral region. Nano Lett., 18, 4584-4589(2018).
[45] Y. Chen, X. Yang, J. Gao. 3D Janus plasmonic helical nanoapertures for polarization-encrypted data storage. Light Sci. Appl., 8, 45(2019).
[46] K. Chen, G. Ding, G. Hu, Z. Jin, J. Zhao, Y. Feng, T. Jiang, A. Alù, C. W. Qiu. Directional Janus metasurface. Adv. Mater., 32, 1906352(2020).
[47] Y. Su, Y. Li, T. Yang, T. Han, Y. Sun, J. Xiong, L. Wu, C. W. Qiu. Path-dependent thermal metadevice beyond Janus functionalities. Adv. Mater., 33, 2003084(2021).
[48] H. Lu, E. Thelander, J. W. Gerlach, U. Decker, B. Zhu, B. Rauschenbach. Single pulse laser-induced phase transitions of PLD-deposited Ge2Sb2Te5 films. Adv. Funct. Mater., 23, 3621-3627(2013).
[49] D. Chiang, T.-R. Jeng, D.-R. Huang, Y.-Y. Chang, C.-P. Liu. Kinetic crystallization behavior of phase-change medium. Jpn. J. Appl. Phys., 38, 1649-1651(1999).
[50] S. Yu, L. Li, G. Shi, C. Zhu, Y. Shi. Generating multiple orbital angular momentum vortex beams using a metasurface in radio frequency domain. Appl. Phys. Lett., 108, 241901(2016).
[51] S. Tang, T. Cai, G.-M. Wang, J.-G. Liang, X. Li, J. Yu. High-efficiency dual-modes vortex beam generator with polarization-dependent transmission and reflection properties. Sci. Rep., 8, 6422(2018).
[52] S. Raoux, F. Xiong, M. Wuttig, E. Pop. Phase change materials and phase change memory. MRS Bull., 39, 703-710(2014).
[53] A. Pirovano, A. Redaelli, F. Pellizzer, F. Ottogalli, M. Tosi, D. Ielmini, A. L. Lacaita, R. Bez. Reliability study of phase-change nonvolatile memories. IEEE Trans. Device Mater. Reliab., 4, 422-427(2004).
[54] R. E. Simpson, P. Fons, A. V. Kolobov, T. Fukaya, M. Krbal, T. Yagi, J. Tominaga. Interfacial phase-change memory. Nat. Nanotechnol., 6, 501-505(2011).
[55] S.-H. Lee, Y. Jung, R. Agarwal. Highly scalable non-volatile and ultra-low-power phase-change nanowire memory. Nat. Nanotechnol., 2, 626-630(2007).
[56] I. Kim, S. Cho, D. Im, E. Cho, D. Kim, G. Oh, D. Ahn, S. Park, S. Nam, J. Moon. High performance PRAM cell scalable to sub-20 nm technology with below 4F2 cell size, extendable to DRAM applications. Symposium on VLSI Technology, 203-204(2010).
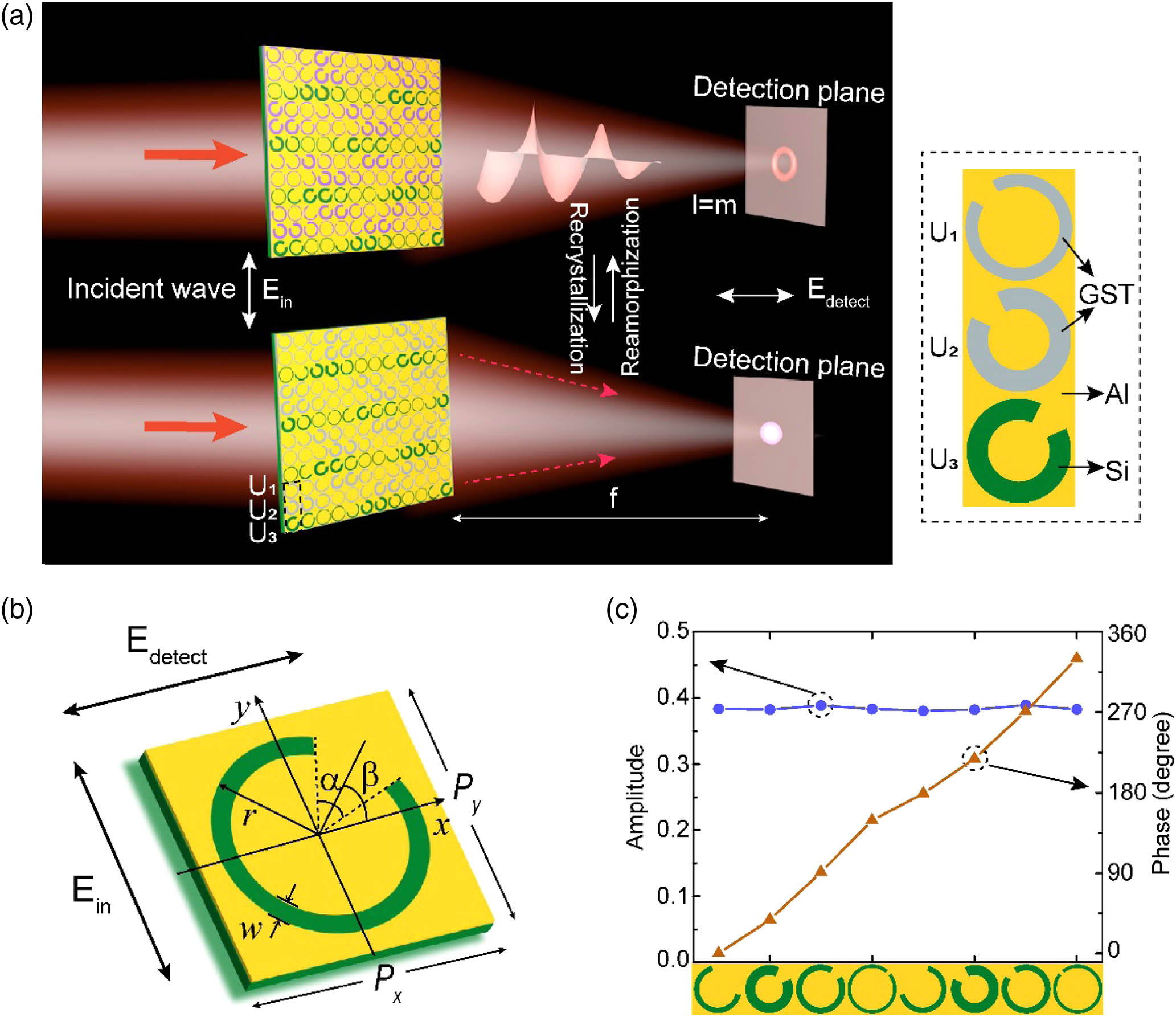
Set citation alerts for the article
Please enter your email address