
- Journal of Semiconductors
- Vol. 43, Issue 2, 021801 (2022)
Abstract
1. Introduction
Diamond has an extraordinary combination of outstanding characteristics, making it an 'extreme' material highly desirable for various applications. Due to its well-known mechanical properties, in particular hardness, it is extensively used as an abrasive and tooling material in a wide variety of industrial applications, including indenters, grinding, cutting, drilling, polishing, honing, sharpening, lapping, sanding, and wire drawing tools, usually in high-stress conditions. Additionally, they are responsible for the ability of diamond anvil cells with diamond compacts for high-pressure, high-temperature research[
Despite its outstanding features for electronics and photonic devices, considerable improvements are still anticipated since its present level is far lower. Its operation lifespan is far shorter than projected, and it has remained at the fundamental research level for decades. Additionally, reproducible and large-scale manufacture of diamond crystals continues to be a challenge[
2. Diamond electronic devices and nano- or micro-electromechanical systems
Diamond is an enthralling semiconductor with extraordinary physical features, including ultrawide bandgap (5.47 eV), ultra-high thermal conductivity (2200 W/(m·K))[
2.1. Diamond diodes
The most often used semiconductor diode is a crystalline semiconductor material coupled to two electrical terminals through a p–n junction. For high-power and microwave switching, diodes serve as the basic structure, and they also serve as the building blocks for three-terminal devices. Both unipolar and bipolar diodes have been experimentally demonstrated, including p-type-intrinsic-n-type (p–i–n) diodes, Schottky barrier diode (SBD)[
Figure 1.(Color online) Representative diamond devices. (a, b) A picture and schematic of a diamond SBD[
2.2. Field-effect transistors
Diamond has been investigated in the exploitation of prototype field-effect transistors (FETs). At the moment, several designs for power electronics applications which is suitable to high temperature and radiation settings are being investigated. Lee et al.[
2.3. Diamond nano- or micro-electromechanical systems
NEMS/MEMS have attracted interest in various applications, including accelerometers, physical, biological, and chemical sensors. Diamond has demonstrated itself as an ideal candidate for developing robust, high-reliable, and long-endurance NEMS/MEMS in harsh environments due to its excellent physical, chemical, electrical, and tribomechanical characteristics[
Additionally, theoretical proposals and fundamental experiments to mechanically couple NEMS/MEMS devices to intrinsic quantum two-level systems can be achieved in a diamond nano-mechanical resonator of embedded nitrogen-vacancy (NV) centers[
2.4. Microfabrication techniques of diamond devices
Fabrication of diamond devices and NEMS/MEMS is often challenging, in particular for single-crystalline diamond (SCD), since they can only be from homoepitaxially/heteroepitaxial growth on previously grown SCD or other substrates. As a result, the accessible SCD templates are just a few tens of millimeters square. Manufacturing diamond devices and NEMS/MEMS typically begins with the fabrication of a diamond thin film or membrane, which is then followed by the assembly of the devices. Methods have been investigated that include the “thin down” technique, the liftoff method, the angle-etching procedure, and focused ion beam milling[
The “thin down” technique begins with a bulk diamond with a thickness of tens of microns. It is subsequently thinned down to a thickness of a few hundred nanometers through dry etching by capacitively coupled reactive ion etching (RIE)[
3. Challenges on scalable diamond devices
For mass production of industrial semiconductor devices, semiconductor materials are required to achieve high purity, low defect density, high carrier concentration and mobility, and large area wafer. However, doping and fabrication of high-quality large-area SCD wafers are still worldwide scientific problems for diamond materials. The tremendous potential for realizing diamond devices above present device technology is contingent upon achieving several key objectives, including the synthesis of ultrahigh purity, high-quality wafer-scale SCDs, and the ability to provide carriers via controlled doping.
3.1. Growth of large-area single-crystalline diamond wafers
Although commercially accessible high-quality polycrystalline diamond has many attributes comparable to the finest natural diamonds, grain boundaries can still impact its electronic performances due to the crystal defect nature and existence of various impurities such as nitrogen, silicon, and others[
For the past 70 years, synthetic SCDs have been manufactured using high-pressure, high-temperature (HPHT) processes[
Numerous approaches to large-area SCD wafer (Figs. 2(a)–2(c)) synthesis reach 50 mm in diameter. In homoepitaxy, single crystals, such as those obtained by the HPHT method, are utilized as seeds and are replicated namely "mosaic" wafer. Among the numerous techniques for CVD diamond, microwave plasma CVD has attracted significant attention owing to its ability to combine high purity with rapid growth rates (>100μm/h)[
Figure 2.(Color online) Synthetic diamonds. (a) Microwave plasma-assisted CVD growth of SCD over 70 large 3.5 × 3.5 mm2 HPHT seed crystals[
In heteroepitaxy, the substrate is critical for the SCD's properties: easy acquisition of high-quality and large-size substrates, the substrate's lattice mismatch with diamond to reduce misfit dislocations at the interface, excellent thermal expansion coefficient matching with diamond to reduce thermal stress, carbon's solubility in the substrate, and the interaction with C atoms (specifically, its inclination to produce stable carbides) all affect the nucleation and growth of diamond. c-BN[
Nevertheless, due to the scarcity of single-crystalline Ir substrates, Ir multilayer substrates (such as Ir/MgO[
3.2. Doping of diamond
Several issues should be fixed to fully use diamond's electrical capabilities before this material can be used largely. The primary impediment[
3.2.1. p-type diamond
Boron, one less electron than carbon, is very easy to integrate into diamond due to its tiny atomic radius[
3.2.2. n-type diamond
On the other hand, n-type diamond does not occur naturally, and it is challenging to acquire n-type semiconducting diamond with high quality. Since the early 1980s, when the CVD method for the growth of diamond was developed, n-type doping has been studied. Based on the conventional dopants used in Si, it is anticipated that group V (N, P) and VI (S) elements in diamond are promising donor impurities. Nitrogen (with its extra electron compared to C) is the most common impurity in an n-type diamond. Nitrogen exists in diamonds in alternative ways, and substitutional nitrogen easily enters the diamond lattice, which fits in with its negative formation energy (–3.4 eV)[
The poor phosphorus incorporation efficiency is still a serious obstacle, although Ohtani et al.[
4. Elastic strain engineering of diamond
Elastic strain engineering (ESE) can bring materials with unique characteristics by applying considerable mechanical stress or strain[
4.1. Elastic deformation of diamond
In past decades, the mechanical properties of diamond have been investigated using various techniques such as indentation[
Figure 3.(Color online) Elastic strain engineering of diamond. (a–c) Schematic of the "push-to-bend" deformation of the nanoscale diamond needle with maximum deformation just before fracture, corresponding FEM simulation reproducing the geometry of the bending and displaying the local elastic maximum principal strain[
4.2. Uniform tensile elastic straining of diamond
With the improved microfabrication techniques, as shown in Fig. 3(e), single-crystalline diamond bridge structures can be precisely microfabricated by FIB-assist processes, and a uniaxial tensile test can be realized for such smooth diamond specimens by using a nanoindenter with a customized tensile diamond gripper[
4.3. Tuning electronic properties of strained diamonds
The experimental discoveries have established that the diamond can be elastically deformed up to its theoretical strain limits. These advances offer possibilities to explore its functional performances by elastic strain engineering. A surprising atomistic ductility and electronic conductivity were revealed in diamonds under complex loading conditions of large concurrent shear and compressive strains by density functional theory (DFT) calculation[
5. Conclusions and perspectives
With the increasing need for wide bandgap semiconductors, the arrival of 5G and even future 6G networks, and the demand for power electronics chips for electric vehicles, diamond has become an ideal electronic material for such critical applications. Here, we can only briefly cover a few efforts on diamond-based high voltage switching diodes, high-power high-frequency FET, devices operating at high temperatures, and MEMS/NEMS. Although diamond offers enormous potential, it also has several characteristics that pose difficulties. We then described the production issues, including the wafer-scale production of electronics-grade high-quality diamonds. Significant progress has been made on high-quality CVD diamond thin films with a 4-inch diameter[
We also described the shallow doping problems in diamonds for realizing diamond microelectronics applications. The growth and application of p-type diamonds with boron-doping are considerably mature. Ion implantation or CVD may be used to precisely control the impurity level and carrier transport performance. The absence of effective and practical n-type conductive diamond materials, on the other hand, restricts the use of diamond semiconductor materials in the electronic field. In general, there are still great difficulties in the synthesis of low-resistivity n-type diamond films. It deserves further investigation into improving doping technology, increasing electron mobility, and reducing resistivity in the fabrication of n-type diamond semiconductors. We then proposed an alternative way, “elastic strain engineering”, to modulate a diamond’s electronic properties, which was considered impossible due to its inherent mechanical characteristics. Now with the development of microfabrication techniques, ultralarge elastic strains can be well realized in diamond micro/nanostructures[
Additionally, as diamond is also a popular quantum material[
Nevertheless, grand challenges remain in permitting diamond microelectronics and optoelectronics to the industry level. For example, establishing the planar processing of diamond for high-efficiency and reproducible large-scale manufacturing would be the primary challenge for building individual components of integrated circuit chips. Besides, maintaining the robust, highly strained diamond for an extended length of time with accurate bandgap control and characterization would be another challenge. A lattice mismatch of diamond films grown on different heterogeneous substrates could be a possible way to realize certain “strained diamond” devices and ultimately incorporate them into large-scale productions, but deep ESE requires some fundamentally new strategies and manufacturing processes for practical implementation of strained diamond architectures. This exciting field is currently undergoing rapid development and definitely needs joint efforts from multi-disciplinary researchers and industrial experts.
Acknowledgements
We acknowledge the support from the Research Grants Council of the Hong Kong Special Administrative Region, China (Grant RFS2021-1S05), and the National Natural Science Foundation of China (Grant 11922215). H.Z. acknowledges the funding from the National Natural Science Foundation of China (Grant 11902200) and the Science and Technology Commission of Shanghai Municipality (Grant 19YF1433600).
References
[1] A Jayaraman. Diamond anvil cell and high-pressure physical investigations. Rev Mod Phys, 55, 65(1983).
[2] B Li, C Ji, W Yang et al. Diamond anvil cell behavior up to 4 Mbar. PNAS, 115, 1713(2018).
[3] J Xia, J X Yan, Z H Wang et al. Strong coupling and pressure engineering in WSe2–MoSe2 heterobilayers. Nat Phys, 17, 92(2021).
[4] P W May. The new diamond age. Science, 319, 1490(2008).
[5] C J H Wort, R S Balmer. Diamond as an electronic material. Mater Today, 11, 22(2008).
[6] I Aharonovich, A D Greentree, S Prawer. Diamond photonics. Nat Photonics, 5, 397(2011).
[7] H Watanabe, C E Nebel, S Shikata. Isotopic homojunction band engineering from diamond. Science, 324, 1425(2009).
[8] J Y Tsao, S Chowdhury, M A Hollis et al. Ultrawide-bandgap semiconductors: Research opportunities and challenges. Adv Electron Mater, 4, 1600501(2018).
[9] J E Field. The properties of natural and synthetic diamond. Academic Press(1992).
[10] J Isberg, J Hammersberg, E Johansson et al. High carrier mobility in single-crystal plasma-deposited diamond. Science, 297, 1670(2002).
[11] M Schreck, J Asmussen, S Shikata et al. Large-area high-quality single crystal diamond. MRS Bull, 39, 504(2014).
[12] I Friel, S L Clewes, H K Dhillon et al. Control of surface and bulk crystalline quality in single crystal diamond grown by chemical vapour deposition. Diam Relat Mater, 18, 808(2009).
[13] A Tallaire, O Brinza, V Mille et al. Reduction of dislocations in single crystal diamond by lateral growth over a macroscopic hole. Adv Mater, 29, 1604823(2017).
[14] Y J Lu, C N Lin, C X Shan. Optoelectronic diamond: Growth, properties, and photodetection applications. Adv Opt Mater, 6, 1800359(2018).
[15] L Reggiani, S Bosi, C Canali et al. Hole-drift velocity in natural diamond. Phys Rev B, 23, 3050(1981).
[16] G Perez, A Maréchal, G Chicot et al. Diamond semiconductor performances in power electronics applications. Diam Relat Mater, 110, 108154(2020).
[17] K Miyata, D L Dreifus, K Kobashi. Metal-intrinsic semiconductor-semiconductor structures using polycrystalline diamond films. Appl Phys Lett, 60, 480(1992).
[18] K Miyata, K Kobashi, D L Dreifus. Rectifying diodes with a metal/intrinsic semiconductor/semiconductor structure using polycrystalline diamond films. Diam Relat Mater, 2, 1107(1993).
[19] M Brezeanu, S J Rashid, G A J Amaratunga et al. On-state behaviour of diamond M-i-P structures. 2006 International Semiconductor Conference, 311(2006).
[20] P N Volpe, P Muret, J Pernot et al. Extreme dielectric strength in boron doped homoepitaxial diamond. Appl Phys Lett, 97, 223501(2010).
[21] A Traoré, A Nakajima, T Makino et al. Reverse-recovery of diamond p-i-n diodes. IET Power Electron, 11, 695(2018).
[22] A Traoré, P Muret, A Fiori et al. Zr/oxidized diamond interface for high power Schottky diodes. Appl Phys Lett, 104, 052105(2014).
[23] V S Bormashov, S A Terentiev, S G Buga et al. Thin large area vertical Schottky barrier diamond diodes with low on-resistance made by ion-beam assisted lift-off technique. Diam Relat Mater, 75, 78(2017).
[24] H Umezawa, S I Shikata, T Funaki. Diamond Schottky barrier diode for high-temperature, high-power, and fast switching applications. Jpn J Appl Phys, 53, 05FP06(2014).
[25] T Shimaoka, H Umezawa, K Ichikawa et al. Ultrahigh conversion efficiency of betavoltaic cell using diamond pn junction. Appl Phys Lett, 117, 103902(2020).
[26] Y T Lee, A Vardi, M Tordjman. A hybrid self-aligned MIS-MESFET architecture for improved diamond-based transistors. Appl Phys Lett, 117, 202101(2020).
[27] F Maier, M Riedel, B Mantel et al. Origin of surface conductivity in diamond. Phys Rev Lett, 85, 3472(2000).
[28] J L Liu, H Yu, S W Shao et al. Carrier mobility enhancement on the H-terminated diamond surface. Diam Relat Mater, 104, 107750(2020).
[29] N J Yang, S Y Yu, J V MacPherson et al. Conductive diamond: Synthesis, properties, and electrochemical applications. Chem Soc Rev, 48, 157(2019).
[30] M H Zhang, W Wang, G Q Chen et al. Electrical properties of yttrium gate hydrogen-terminated diamond field effect transistor with Al2O3 dielectric layer. Appl Phys Lett, 118, 053506(2021).
[31] T Matsumoto, H Kato, K Oyama et al. Inversion channel diamond metal-oxide-semiconductor field-effect transistor with normally off characteristics. Sci Rep, 6, 31585(2016).
[32] C Masante, N Rouger, J Pernot. Recent progress in deep-depletion diamond metal–oxide–semiconductor field-effect transistors. J Phys D, 54, 233002(2021).
[33] C Masante, J Pernot, A Maréchal et al. High temperature operation of a monolithic bidirectional diamond switch. Diam Relat Mater, 111, 108185(2021).
[34] E Kohn, P Gluche, M Adamschik. Diamond MEMS—a new emerging technology. Diam Relat Mater, 8, 934(1999).
[35] A V Sumant, O Auciello, R W Carpick et al. Ultrananocrystalline and nanocrystalline diamond thin films for MEMS/NEMS applications. MRS Bull, 35, 281(2010).
[36] M Possas-Abreu, L Rousseau, F Ghassemi et al. Biomimetic diamond MEMS sensors based on odorant-binding proteins: Sensors validation through an autonomous electronic system. 2017 ISOCS/IEEE International Symposium on Olfaction and Electronic Nose (ISOEN), 1(2017).
[37] M Y Liao, L W Sang, T Teraji et al. Ultrahigh performance on-chip single crystal diamond NEMS/MEMS with electrically tailored self-sensing enhancing actuation. Adv Mater Technol, 4, 1800325(2019).
[38] M Y Liao. Progress in semiconductor diamond photodetectors and MEMS sensors. Funct Diam, 1, 29(2021).
[39] O Auciello, D M Aslam. Review on advances in microcrystalline, nanocrystalline and ultrananocrystalline diamond films-based micro/nano-electromechanical systems technologies. J Mater Sci, 56, 7171(2021).
[40] Y Tao, J M Boss, B A Moores et al. Single-crystal diamond nanomechanical resonators with quality factors exceeding one million. Nat Commun, 5, 3638(2014).
[41] P Rath, S Khasminskaya, C Nebel et al. Diamond-integrated optomechanical circuits. Nat Commun, 4, 1690(2013).
[42] P Rath, S Ummethala, S Diewald et al. Diamond electro-optomechanical resonators integrated in nanophotonic circuits. Appl Phys Lett, 105, 251102(2014).
[43] A Pályi, P R Struck, M Rudner et al. Spin-orbit-induced strong coupling of a single spin to a nanomechanical resonator. Phys Rev Lett, 108, 206811(2012).
[44] I Wilson-Rae, P Zoller, A Imamoğlu. Laser cooling of a nanomechanical resonator mode to its quantum ground state. Phys Rev Lett, 92, 075507(2004).
[45] J Teissier, A Barfuss, P Appel et al. Strain coupling of a nitrogen-vacancy center spin to a diamond mechanical oscillator. Phys Rev Lett, 113, 020503(2014).
[46] A Barfuss, J Teissier, E Neu et al. Strong mechanical driving of a single electron spin. Nat Phys, 11, 820(2015).
[47] J Riedrich-Möller, L Kipfstuhl, C Hepp et al. One- and two-dimensional photonic crystal microcavities in single crystal diamond. Nat Nanotechnol, 7, 69(2012).
[48] P Rath, S Ummethala, C Nebel et al. Diamond as a material for monolithically integrated optical and optomechanical devices. Phys Status Solidi A, 212, 2385(2015).
[49] D Rani, O Opaluch, E Neu. Recent advances in single crystal diamond device fabrication for photonics, sensing and nanomechanics. Micromachines, 12, 36(2020).
[50] A H Piracha, P Rath, K Ganesan et al. Scalable fabrication of integrated nanophotonic circuits on arrays of thin single crystal diamond membrane windows. Nano Lett, 16, 3341(2016).
[51] Y Tao, C Degen. Facile fabrication of single-crystal-diamond nanostructures with ultrahigh aspect ratio. Adv Mater, 25, 3962(2013).
[52] N R Parikh, J D Hunn, E McGucken et al. Single-crystal diamond plate liftoff achieved by ion implantation and subsequent annealing. Appl Phys Lett, 61, 3124(1992).
[53] B A Fairchild, P Olivero, S Rubanov et al. Fabrication of ultrathin single-crystal diamond membranes. Adv Mater, 20, 4793(2008).
[54] M Y Liao, C Li, S Hishita et al. Batch production of single-crystal diamond bridges and cantilevers for microelectromechanical systems. J Micromech Microeng, 20, 085002(2010).
[55] H A Atikian, P Latawiec, M J Burek et al. Freestanding nanostructures via reactive ion beam angled etching. APL Photonics, 2, 051301(2017).
[56] I Bayn, S Mouradian, L Li et al. Fabrication of triangular nanobeam waveguide networks in bulk diamond using single-crystal silicon hard masks. Appl Phys Lett, 105, 211101(2014).
[57] M K Zalalutdinov, M P Ray, D M Photiadis et al. Ultrathin single crystal diamond nanomechanical dome resonators. Nano Lett, 11, 4304(2011).
[58] W R McKenzie, M Z Quadir, M H Gass et al. Focused Ion beam implantation of diamond. Diam Relat Mater, 20, 1125(2011).
[59] S Rubanov, A Suvorova. Ion implantation in diamond using 30 keV Ga+ focused ion beam. Diam Relat Mater, 20, 1160(2011).
[60] Z Tong, X C Luo. Investigation of focused ion beam induced damage in single crystal diamond tools. Appl Surf Sci, 347, 727(2015).
[61] I Bayn, A Bolker, C Cytermann et al. Diamond processing by focused ion beam—surface damage and recovery. Appl Phys Lett, 99, 183109(2011).
[62] P Němec, J Preclíková, A Kromka et al. Ultrafast dynamics of photoexcited charge carriers in nanocrystalline diamond. Appl Phys Lett, 93, 083102(2008).
[63] C Fang, Y W Zhang, Z F Zhang et al. Preparation of “natural” diamonds by HPHT annealing of synthetic diamonds. CrystEngComm, 20, 505(2018).
[64] F P Bundy, H T Hall, H M Strong et al. Man-made diamonds. Nature, 176, 51(1955).
[65] H T Hall. Sintered diamond: A synthetic carbonado. Science, 169, 868(1970).
[66] L W Yin, N W Wang, Z D Zou et al. Formation and crystal structure of metallic inclusions in a HPHT as-grown diamond single crystal. Appl Phys A, 71, 473(2000).
[67] J C Angus, H A Will, W S Stanko. Growth of diamond seed crystals by vapor deposition. J Appl Phys, 39, 2915(1968).
[68] G Y Shu, B Dai, V G Ralchenko et al. Vertical-substrate epitaxial growth of single-crystal diamond by microwave plasma-assisted chemical vapor deposition. J Cryst Growth, 486, 104(2018).
[69] G Shu, B Dai, V G Ralchenko et al. Growth of three-dimensional diamond mosaics by microwave plasma-assisted chemical vapor deposition. CrystEngComm, 20, 198(2018).
[70] R C Burns, A I Chumakov, S H Connell et al. HPHT growth and X-ray characterization of high-quality type IIa diamond. J Phys Condens Matter, 21, 364224(2009).
[71] S N Polyakov, V N Denisov, V Kuzmin N et al. Characterization of top-quality type IIa synthetic diamonds for new X-ray optics. Diam Relat Mater, 20, 726(2011).
[72] V Yurov, E Bushuev, A Bolshakov et al. Etching kinetics of (100) single crystal diamond surfaces in a hydrogen microwave plasma, studied with In Situ low-coherence interferometry. Phys Status Solidi A, 214, 1700177(2017).
[73] Q Liang, C Y Chin, J Lai et al. Enhanced growth of high quality single crystal diamond by microwave plasma assisted chemical vapor deposition at high gas pressures. Appl Phys Lett, 94, 024103(2009).
[74] M Füner, C Wild, P Koidl. Novel microwave plasma reactor for diamond synthesis. Appl Phys Lett, 72, 1149(1998).
[75] H Yamada, A Chayahara, Y Mokuno et al. A 2-in. mosaic wafer made of a single-crystal diamond. Appl Phys Lett, 104, 102110(2014).
[76] M Schreck, S Gsell, R Brescia et al. Ion bombardment induced buried lateral growth: The key mechanism for the synthesis of single crystal diamond wafers. Sci Rep, 7, 44462(2017).
[77] S Ohmagari, H Yamada, N Tsubouchi et al. Schottky barrier diodes fabricated on diamond mosaic wafers: Dislocation reduction to mitigate the effect of coalescence boundaries. Appl Phys Lett, 114, 082104(2019).
[78] A Argoitia, J C Angus, J S Ma et al. Heteroepitaxy of diamond on c-BN: Growth mechanisms and defect characterization. J Mater Res, 9, 1849(1994).
[79] L Wang, P Pirouz, A Argoitia et al. Heteroepitaxially grown diamond on a c-BN {111} surface. Appl Phys Lett, 63, 1336(1993).
[80] T Tachibana, Y Yokota, K Miyata et al. Diamond films heteroepitaxially grown on platinum (111). Phys Rev B, 56, 15967(1997).
[81] W Zhu, P C Yang, J T Glass. Oriented diamond films grown on nickel substrates. Appl Phys Lett, 63, 1640(1993).
[82] W Liu, D A Tucker, P C Yang et al. Nucleation of oriented diamond particles on cobalt substrates. J Appl Phys, 78, 1291(1995).
[83] X Jiang, C P Klages. Heteroepitaxial diamond growth on (100) silicon. Diam Relat Mater, 2, 1112(1993).
[84] H Kawarada, C Wild, N Herres et al. Heteroepitaxial growth of highly oriented diamond on cubic silicon carbide. J Appl Phys, 81, 3490(1997).
[85] S D Wolter, M T McClure, J T Glass et al. Bias-enhanced nucleation of highly oriented diamond on titanium carbide (111) substrates. Appl Phys Lett, 66, 2810(1995).
[86] H Bensalah, I Stenger, G Sakr et al. Mosaicity, dislocations and strain in heteroepitaxial diamond grown on iridium. Diam Relat Mater, 66, 188(2016).
[87] K Ichikawa, K Kurone, H Kodama et al. High crystalline quality heteroepitaxial diamond using grid-patterned nucleation and growth on Ir. Diam Relat Mater, 94, 92(2019).
[88] K Ohtsuka, K Suzuki, A Sawabe et al. Epitaxial growth of diamond on iridium. Jpn J Appl Phys, 35, L1072(1996).
[89] M Schreck, T Bauer, S Gsell et al. Domain formation in diamond nucleation on iridium. Diam Relat Mater, 12, 262(2003).
[90] M J Verstraete, J C Charlier. Why is iridium the best substrate for single crystal diamond growth. Appl Phys Lett, 86, 191917(2005).
[91] S Kono, M Shiraishi, N I Plusnin et al. X-ray photoelectron diffraction study of the initial stages of CVD diamond heteroepitaxy on Ir (001)/SrTiO3. New Diam Front Carbon Technol, 15, 363(2005).
[92] N Vaissiere, S Saada, M Bouttemy et al. Heteroepitaxial diamond on iridium: New insights on domain formation. Diam Relat Mater, 36, 16(2013).
[93] S Washiyama, S Mita, K Suzuki et al. Coalescence of epitaxial lateral overgrowth-diamond on stripe-patterned nucleation on Ir/MgO(001). Appl Phys Express, 4, 095502(2011).
[94] T Fujisaki, M Tachiki, N Taniyama et al. Initial growth of heteroepitaxial diamond on Ir (001)/MgO (001) substrates using antenna-edge-type microwave plasma assisted chemical vapor deposition. Diam Relat Mater, 12, 246(2003).
[95] S W Kim, Y Kawamata, R Takaya et al. Growth of high-quality one-inch free-standing heteroepitaxial (001) diamond on (11-20) sapphire substrate. Appl Phys Lett, 117, 202102(2020).
[96] A Samoto, S Ito, A Hotta et al. Investigation of heterostructure between diamond and iridium on sapphire. Diam Relat Mater, 17, 1039(2008).
[97] C Bednarski, Z Dai, A P Li et al. Studies of heteroepitaxial growth of diamond. Diam Relat Mater, 12, 241(2003).
[98] K H Lee, S Saada, J C Arnault et al. Epitaxy of iridium on SrTiO3/Si (001): A promising scalable substrate for diamond heteroepitaxy. Diam Relat Mater, 66, 67(2016).
[99] T Bauer, S Gsell, M Schreck et al. Growth of epitaxial diamond on silicon via iridium/SrTiO3 buffer layers. Diam Relat Mater, 14, 314(2005).
[100] M Fischer, R Brescia, S Gsell et al. Growth of twin-free heteroepitaxial diamond on Ir/YSZ/Si(111). J Appl Phys, 104, 123531(2008).
[101] M Regmi, K More, G Eres. A narrow biasing window for high density diamond nucleation on Ir/YSZ/Si(100) using microwave plasma chemical vapor deposition. Diam Relat Mater, 23, 28(2012).
[102] S T Lee, Y Lifshitz. The road to diamond wafers. Nature, 424, 500(2003).
[103] S Gsell, T Bauer, J Goldfuß et al. A route to diamond wafers by epitaxial deposition on silicon via iridium/yttria-stabilized zirconia buffer layers. Appl Phys Lett, 84, 4541(2004).
[104] S S Zhang, Z H Li, K Luo et al. Discovery of carbon-based strongest and hardest amorphous material. Natl Sci Rev, in press(2021).
[105] S S Zhang, Y J Wu, K Luo et al. Narrow-gap, semiconducting, superhard amorphous carbon with high toughness, derived from C60 fullerene. Cell Rep Phys Sci, 2, 100575(2021).
[106] J Asmussen, T A Grotjohn, T Schuelke et al. Multiple substrate microwave plasma-assisted chemical vapor deposition single crystal diamond synthesis. Appl Phys Lett, 93, 031502(2008).
[107] T P Chow, R Tyagi. Wide bandgap compound semiconductors for superior high-voltage unipolar power devices. IEEE Trans Electron Devices, 41, 1481(1994).
[108] D B Laks, C G van de Walle, G F Neumark et al. Role of native defects in wide-band-gap semiconductors. Phys Rev Lett, 66, 648(1991).
[109] M Nesladek. Conventional n-type doping in diamond: State of the art and recent progress. Semicond Sci Technol, 20, R19(2005).
[110] T H Borst, O Weis. Boron-doped homoepitaxial diamond layers: Fabrication, characterization, and electronic applications. Phys Status Solidi A, 154, 423(1996).
[111] R Kalish. The search for donors in diamond. Diam Relat Mater, 10, 1749(2001).
[112] R M Chrenko. Boron, the dominant acceptor in semiconducting diamond. Phys Rev B, 7, 4560(1973).
[113] Z Teukam, J Chevallier, C Saguy et al. Shallow donors with high n-type electrical conductivity in homoepitaxial deuterated boron-doped diamond layers. Nat Mater, 2, 482(2003).
[114] E A Ekimov, V A Sidorov, E D Bauer et al. Superconductivity in diamond. Nature, 428, 542(2004).
[115] L Boeri, J Kortus, O K Andersen. Three-dimensional MgB2-type superconductivity in hole-doped diamond. Phys Rev Lett, 93, 237002(2004).
[116] K W Lee, W E Pickett. Superconductivity in boron-doped diamond. Phys Rev Lett, 93, 237003(2004).
[117] H J Xiang, Z Y Li, J L Yang et al. Electron-phonon coupling in a boron-doped diamond superconductor. Phys Rev B, 70, 212504(2004).
[118] X Blase, C Adessi, D Connétable. Role of the dopant in the superconductivity of diamond. Phys Rev Lett, 93, 237004(2004).
[119] Y M Ma, J S Tse, T Cui et al. First-principles study of electron-phonon coupling in hole- and electron-doped diamonds in the virtual crystal approximation. Phys Rev B, 72, 014306(2005).
[120] F Giustino, J R Yates, I Souza et al. Electron-phonon interaction via electronic and lattice wannier functions: Superconductivity in boron-doped diamond reexamined. Phys Rev Lett, 98, 047005(2007).
[121] A Kawano, H Ishiwata, S Iriyama et al. Superconductor-to-insulator transition in boron-doped diamond films grown using chemical vapor deposition. Phys Rev B, 82, 085318(2010).
[122] F Lloret, D Eon, E Bustarret et al. Selectively boron doped homoepitaxial diamond growth for power device applications. Appl Phys Lett, 118, 023504(2021).
[123] K Tsukioka, H Okushi. Hall mobility and scattering mechanism of holes in boron-doped homoepitaxial chemical vapor deposition diamond thin films. Jpn J Appl Phys, 45, 8571(2006).
[124] V S Bormashov, S A Tarelkin, S G Buga et al. Electrical properties of the high quality boron-doped synthetic single-crystal diamonds grown by the temperature gradient method. Diam Relat Mater, 35, 19(2013).
[125] J Isberg, A Lindblom, A Tajani et al. Temperature dependence of hole drift mobility in high-purity single-crystal CVD diamond. Phys Status Solidi A, 202, 2194(2005).
[126] S A Kajihara, A Antonelli, J Bernholc et al. Nitrogen and potentialn-type dopants in diamond. Phys Rev Lett, 66, 2010(1991).
[127] S Koizumi, M Kamo, Y Sato et al. Growth and characterization of phosphorus doped n-type diamond thin films. Diam Relat Mater, 7, 540(1998).
[128] R Kalish. Doping of diamond. Carbon, 37, 781(1999).
[129] S Koizumi, T Teraji, H Kanda. Phosphorus-doped chemical vapor deposition of diamond. Diam Relat Mater, 9, 935(2000).
[130] E Gheeraert, S Koizumi, T Teraji et al. Electronic transitions of electrons bound to phosphorus donors in diamond. Solid State Commun, 113, 577(2000).
[131] M Nesládek, K Meykens, K Haenen et al. Photocurrent and optical absorption spectroscopic study of n-type phosphorus-doped CVD diamond. Diam Relat Mater, 8, 882(1999).
[132] S Y Yu, J Xu, H Kato et al. Phosphorus-doped nanocrystalline diamond for supercapacitor application. ChemElectroChem, 6, 1088(2019).
[133] H Kato, S Yamasaki, H Okushi. N-type doping of (001)-oriented single-crystalline diamond by phosphorus. Appl Phys Lett, 86, 222111(2005).
[134] R Ohtani, T Yamamoto, S D Janssens et al. Large improvement of phosphorus incorporation efficiency in n-type chemical vapor deposition of diamond. Appl Phys Lett, 105, 232106(2014).
[135] I Sakaguchi, M N Gamo, Y Kikuchi et al. Sulfur: A donor dopant forn-type diamond semiconductors. Phys Rev B, 60, R2139(1999).
[136] R Kalish, A Reznik, C Uzan-Saguy et al. Is sulfur a donor in diamond. Appl Phys Lett, 76, 757(2000).
[137] D Saada, J Adler, R Kalish. Sulfur: A potential donor in diamond. Appl Phys Lett, 77, 878(2000).
[138] J Li, Z W Shan, E Ma. Elastic strain engineering for unprecedented materials properties. MRS Bull, 39, 108(2014).
[139] J Feng, X F Qian, C W Huang et al. Strain-engineered artificial atom as a broad-spectrum solar energy funnel. Nat Photonics, 6, 866(2012).
[140] P R Chidambaram, C Bowen, S Chakravarthi et al. Fundamentals of silicon material properties for successful exploitation of strain engineering in modern CMOS manufacturing. IEEE Trans Electron Devices, 53, 944(2006).
[141] T Zhu, J Li, S Ogata et al. Mechanics of ultra-strength materials. MRS Bull, 34, 167(2009).
[142] T Zhu, J Li. Ultra-strength materials. Prog Mater Sci, 55, 710(2010).
[143] H T Zhang, J Tersoff, S Xu et al. Approaching the ideal elastic strain limit in silicon nanowires. Sci Adv, 2, e1501382(2016).
[144] P Humble, R H J Hannink. Plastic deformation of diamond at room temperature. Nature, 273, 37(1978).
[145] V Blank, M Popov, G Pivovarov et al. Ultrahard and superhard phases of fullerite C60: Comparison with diamond on hardness and wear. Diam Relat Mater, 7, 427(1998).
[146] M I Eremets, I A Trojan, P Gwaze et al. The strength of diamond. Appl Phys Lett, 87, 141902(2005).
[147] J M Wheeler, R Raghavan, J Wehrs et al. Approaching the limits of strength: Measuring the uniaxial compressive strength of diamond at small scales. Nano Lett, 16, 812(2016).
[148] A Banerjee, D Bernoulli, H Zhang et al. Ultralarge elastic deformation of nanoscale diamond. Science, 360, 300(2018).
[149] Z Shi, E Tsymbalov, M Dao et al. Deep elastic strain engineering of bandgap through machine learning. PNAS, 116, 4117(2019).
[150] C Liu, X Q Song, Q Li et al. Smooth flow in diamond: Atomistic ductility and electronic conductivity. Phys Rev Lett, 123, 195504(2019).
[151] A M Nie, Y Q Bu, P H Li et al. Approaching diamond's theoretical elasticity and strength limits. Nat Commun, 10, 5533(2019).
[152] C Dang, J P Chou, B Dai et al. Achieving large uniform tensile elasticity in microfabricated diamond. Science, 371, 76(2021).
[153] Z Shi, M Dao, E Tsymbalov et al. Metallization of diamond. PNAS, 117, 24634(2020).
[154] C Liu, X Q Song, Q Li et al. Superconductivity in compression-shear deformed diamond. Phys Rev Lett, 124, 147001(2020).
[155] S Yang, Y Wang, D D B Rao et al. High-fidelity transfer and storage of photon states in a single nuclear spin. Nat Photonics, 10, 507(2016).
[156] K Y Yip, K O Ho, K Y Yu et al. Measuring magnetic field texture in correlated electron systems under extreme conditions. Science, 366, 1355(2019).
[157] M V Gustafsson, T Aref, A F Kockum et al. Propagating phonons coupled to an artificial atom. Science, 346, 207(2014).
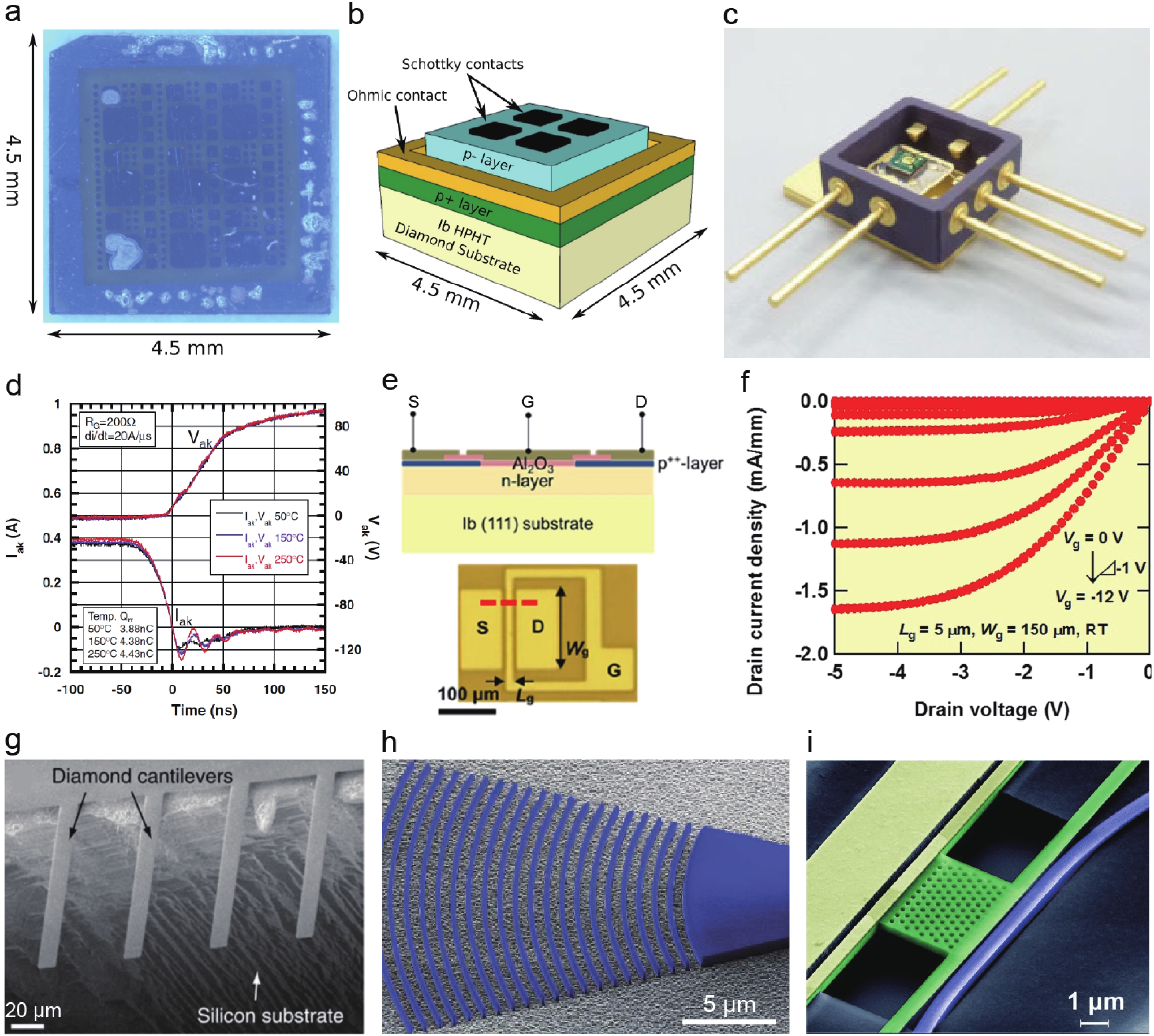
Set citation alerts for the article
Please enter your email address