Abstract
Flexible Bi0.5Sb1.5Te3/epoxy composite thermoelectric films were prepared on polyimide substrates by screen printing. Its electrical transport properties are enhanced by optimizing the content of Bi0.5Sb1.5Te3 powder. The highest power factor of the optimized Bi0.5Sb1.5Te3/epoxy films reached 1.12 mW·m -1·K -2at 300 K, increased by 33% as compared with previous value. The anti-bending test results show that resistance of the thick films remains unchanged when the bending radius is over 20 mm and slightly increases within 3000 bending cycles when the bending radius is 20 mm, implying that the as-prepared films have potential application in flexible TE devices. The flexible thermoelectric leg could establish a temperature difference from 4.2 ℃ to 7.8 ℃ under working current from 0.01 A to 0.05 A, showing potential application in planar cooling field.Development of microelectronic integrated technology resulted in increase in the heat flux density, which seriously affects the performance and service life of the electronic devices[1,2]. Therefore, how to effectively take away the harmful waste heat poses great challenges. Among various heat dissipation techniques[3], thermoelectric (TE) cooling based on Peltier effect of TE materials has attracted increasing attention due to a series of unique advantages such as no noise, no pollution, no moving parts, rapid cooling, simple operation, high reliability, and lightweight, which is expected to solve the problem of heat dissipation of electronic devices with high heat flux[4,5,6]. However, most of the commercial TE cooling devices adopt a vertical design, in which the heat flows along the longitudinal direction[7,8,9]. These TE cooling devices are composed of bulk P and N type thermoelectric legs connected electrically in series through metallic electrodes and then sandwiched between two electrically insulated and thermally conductive ceramic plates. Thus this structure has poor flexibility and is very difficult to realize dimensionally match between TE devices and electronic components[10]. However, the cooling performance deteriorated seriously when the device is downscaled to micro level. While for another type of TE cooling devices using planar design, in which the heat flow is parallel to the substrate, their relatively long TE legs can be fabricated from TE films which are beneficial to flexibility, miniaturization and relatively large temperature difference.
Bi2Te3-based alloys (BixSb2-xTe3 and Bi2Te3-xSex) are customarily regarded as the representative of V2VI3 TE materials, and have been commercialized and widely used for refrigeration and energy conversion applications in the low-temperature range[11,12]. Although great progress was made in developing bulk Bi2Te3 materials with high performance, performance of Bi2Te3-based films is still unsatisfactory. As a simple and efficient process to prepare TE films[13,14,15,16], the bottleneck of printing lies in the deterioration of electrical transport properties which results from low density and various structural defects such as holes and cracks[17]. Several strategies were employed to enhance the electrical transport properties of the printed TE films, such as cold isostatic pressing[18,19], addition of sintering aids[20], and high-temperature sintering to remove the insulating binders[21]. However, realization of high-performance flexible TE cooling devices is still frustrated by the poor electrical transport properties.
In previous work[22], Bi0.5Sb1.5Te3/epoxy flexible thick films with (000l) preferential orientation were prepared on polyimide substrates by combination of brush-printing and hot-pressing curing processes, which greatly improved the electrical transport properties of the composite thick films. However, brush-printing process is difficult to realize mass production due to its poor controllability. In this work, a series of Bi0.5Sb1.5Te3/epoxy composite flexible thermoelectric thick films were successfully prepared on polyimide substrates by screen printing, and the electrical transport properties of the thick films were further improved by optimizing the content of Bi0.5Sb1.5Te3 powder.
1 Experimental
The preparation of thermoelectric slurries refers to the method reported in former work[22]. Thermoelectric slurry was prepared by using diglycidyl ether of bisphenol-F epoxy resin as adhesives, methylhexahydrophthalic anhydride as hardener, 2-ethyl-4-methylimidazole (2E4MI) as anhydride accelerator and Bi0.5Sb1.5Te3 as thermoelectric component. Firstly, Bi0.5Sb1.5Te3 ingots were crushed and pulverized with a planetary ball mill at a speed of 200 r/min for 24 h under the protection of argon, then dried in vacuum oven at 60 ℃ for 2 h to get thermoelectric powder. Secondly, epoxy resin, hardener, catalyst and diluent were mixed to form the epoxy system mixture.
The obtained thermoelectric slurries were printed on the pre-cleaned polyimide (PI) substrates by screen printing to form thick films. The films were dried in vacuum at 100 ℃ for 60 min, and then cured at 300 ℃ for 4 h in hot-pressing apparatus. To further reveal the impact of the content of Bi0.5Sb1.5Te3 on the electrical properties of the composite films, the slurries were prepared with different content of Bi0.5Sb1.5Te3 powder (x=6.5, x=7, x=8, x=9 and x=10) where x represents the mass ratio of Bi0.5Sb1.5Te3 to epoxy system (epoxy resin, hardener and catalyst).
The phase constituents of all thick films were determined by X-ray diffraction (XRD, PANalytical χ’ Pert PRO) using Cu Kα radiation (λ=0.15418 nm). Microstructures were examined by a field emission scanning electron microscope (FESEM, Zeiss ULTRA-PLUS-43-13). The density of the thick films was measured with the Archimedes method. The Hall coefficient (RH), carrier concentration (n), mobility (μH) and electrical conductivity (σ) of the thick films were tested by the HL5500 Hall effect test system at room temperature. The in-plane electrical conductivity (σ) and Seebeck coefficient (α) were measured with the standard four-probe method (Sinkuriko, ZEM-3) in He atmosphere. The measurement error for σ and α is ±5%. The bending tests of the thick films were performed using a homemade bending test apparatus. The temperature distribution of the TE leg was measured under different applied currents (I) by visual infrared thermometer (FLUKE, TI 400).
2 Results and discussion
XRD patterns of these composite films are displayed in Fig. 1. All diffraction peaks can be indexed to the standard diffraction data of Bi0.5Sb1.5Te3 (JCPDS 49- 1713), indicating that these films are composed of single- phase Bi0.5Sb1.5Te3.
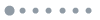
Figure .XRD patterns of the composite films prepared with different contents of Bi0.5Sb1.5Te3 powder
Fig. 2 shows the cross-sectional FESEM images of these composite films. The thicknesses of these films are approximate 20 μm. It is apparent that there are organic residues and pores in all the films. As the content of Bi0.5Sb1.5Te3 powder increased from x=6.5 to x=8, the organic residues were reduced. However, with x further increasing, the pores were obviously increased. Thus the film prepared with x=8 has the most compact microstructure, which could be also identified from the variation of film densities (Table 1).
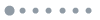
Figure .Cross-sectional FESEM images of the composite thick films prepared with different contents of Bi0.5Sb1.5Te3 powder
x | ρ/(g·cm-3) | RH/(×10-1, cm3·C-1) | n/(×1019, cm-3) | μH/(cm2·V-1·s-1) | σ/(×104, S·m-1) |
---|
6.5 | 3.93 | 3.72 | 1.67 | 45.15 | 1.21 |
7 | 4.08 | 3.69 | 1.69 | 52.17 | 1.41 |
8 | 4.32 | 3.57 | 1.74 | 58.85 | 1.65 |
9 | 4.19 | 3.56 | 1.73 | 54.69 | 1.51 |
10 | 4.05 | 3.55 | 1.72 | 50.59 | 1.40 |
Table 1.
Densities and electrical properties of the composite films at room temperature
The room-temperature electrical transport properties of these composite films are listed in Table 1. The positive Hall coefficient RH indicated that most of carriers were holes. The p-type conduction character was consistent with the corresponding bulk materials[23]. Variation of carrier concentration n with x can be explained by the difference of film density induced by evolution of microstructure. It is noteworthy that the Hall mobility μH of thick films increased by 30% from 45.15 cm2·V-1·s-1 for x=6.5 to 58.85 cm2·V-1·s-1 for x=8. The enhancement of μH is attributed to the improved electrical contact between Bi0.5Sb1.5Te3 powders. However, with x further increasing, the Hall mobility decreased. This is due to the loose microstructure caused by relatively low content of epoxy resin. The variation trend of electrical conductivity σ is consistent with μH.
Fig. 3 shows the temperature dependence of electrical conductivity σ, Seebeck coefficient α and power factor α2σ of these composite films. σ decreased as the test temperature increases, similar with the metallic transport behavior of the bulk Bi0.5Sb1.5Te3 materials[24]. It is worth noting that σ increased significantly with the content of Bi0.5Sb1.5Te3 powder, x increasing from 6.5 to 8 and then decreased as the x was further increased. The improved σ can be explained by the reduced organics and improved grain contact as shown in Fig. 2, which led to greater mobility. When x further increases, the amount of epoxy resin is not enough to effectively adhere the grains, which leads to loose microstructure. Obviously, the deteriorated microstructure must cause decreased μH and σ. As shown in Fig. 3(b), α almost remains constant with x increasing. Thus, the power factor α2σ increased significantly with the content of Bi0.5Sb1.5Te3 powder, x increasing from 6.5 to 8 and then decreased as the x was further increased. When x=8, the optimized power factor of thick films reached 1.12 mW·m-1·K-2. Table 2 summarizes the electrical conductivity σ and power factor α2σ of Bi2Te3-based composite films in this work and literature[10,14,17,20,22,25]. As shown in Table 2, the optimized electrical conductivity and power factor of the as-prepared composite films are much higher than all of the reported composite films, showing increments of 33% and 331% as compared with the power factor of our previous work[22] and Bi2Te3+1%Se/epoxy composite films[20], respectively.
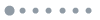
Figure .Temperature dependence of (a) electrical conductivity σ, (b) Seebeck coefficient α and (c) power factor α2σ of the composite thick films prepared with different content of Bi0.5Sb1.5Te3 powder
Materials | Fabrication process | Annealing methods | σ/(×104, S·m-1) | α2σ/(mW·m-1·K-2) | Ref. |
---|
Bi0.5Sb1.5Te3+epoxy | Screen printing | 573 K, 4 h, hot pressing | 1.67 | 1.12 | This work |
Bi0.5Sb1.5Te3 ink | Inkjet printing | 673 K, 30 min | 0.20 | 0.07 | [10] |
Bi0.5Sb1.5Te3+8%Te-epoxy | Dispenser printing | 523 K | 0.11 | 0.06 | [14] |
Bi0.5Sb1.5Te3+C24H44O6 | Brush-printed | 673 K, 4 h | 0.29 | 0.15 | [17] |
Bi2Te3+1%Se-epoxy | Dispenser printing | 623 K, 12 h | 1.03 | 0.26 | [20] |
Bi0.5Sb1.5Te3+epoxy | Brush-printed | 623 K, 4 h, hot pressing | 1.15 | 0.84 | [22] |
Bi0.5Sb1.5Te3+8%Te-polymer | Dispenser printing | 523 K | 0.13 | 0.16 | [25] |
Table 2.
Comparison of σ and α2σ of Bi2Te3-based composite films reported by different groups
Fig. 4 shows the variation rates of resistance (∆R/R0) for the TE leg prepared by the optimal content of Bi0.5Sb1.5Te3 powder before and after anti-bending tests with different bending radius and bending cycles. As shown in Fig. 4(a), the resistance variation rates are almost 0 when the bending radius gradually decreases from 90 mm to 20 mm, and rapidly increases when the bending radius is less than 10 mm. As shown in Fig. 4(b), the resistance variation rates are less than 5% within 3000 bending cycles when the bending radius is 20 mm. The anti-bending test results indicate the as-prepared films have potential application in flexible TE devices.
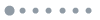
Figure .Anti-bending tests of flexible films: (a) bending radius and (b) bending cycles
Fig. 5 shows the temperature distribution of the TE leg prepared by the optimal content of Bi0.5Sb1.5Te3 powder. As shown in Fig. 5, the TE leg could establish a hot end and a cold end when applied different currents. The temperature difference (DT) between the hot end (Th) and cold end (Tc) increases from 4.2 ℃ to 7.8 ℃ with the current increasing from 0.01 A to 0.05 A, showing potential application in planar cooling field. However, both the temperatures of hot end and cold end gradually increases with the current increasing due to Joule heat effect induced by the internal resistance of the TE leg. According to the thermodynamic analysis of TE cooling device[26,27], there are several different effects for the thermal energy in the device, such as thermal energy from Peltier effect, thermal conduction energy from Fourier effect, and Joule thermal energy from the internal resistance of the device.When the thermal energy from Peltier effect is greater than those from Fourier effect and Joule heat effect, Tc decreases. Thus, the research in next step will focus on decrease of the internal resistance of TE legs and the device structure design.
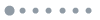
Figure .Visual infrared images of the composite thick films measured with different currents (I)
3 Conclusions
A series of flexible Bi0.5Sb1.5Te3/epoxy composite thermoelectric thick films were successfully prepared on polyimide substrates by screen printing. It is discovered that the content of Bi0.5Sb1.5Te3 powder plays a vital role in improving the microstructure and electrical transport properties. The results indicate that as the content of Bi0.5Sb1.5Te3 powder increases, the electrical conductivity firstly increases and then decreases with the Seebeck coefficient remaining almost constant, and the power factor follows the same trend as the electrical conductivity. The optimized power factor reaches 1.12 mW·K-2·m-1, increased by 33% as compared with our previous work. The anti-bending test indicates that the resistance of the thick films remains unchanged when the bending radius is over 20 mm and slightly increases within 3000 bending cycles when the bending radius is 20 mm, implying that the as-prepared films have potential application in flexible thermoelectric devices. The cooling performance of a single TE leg was evaluated by applying different current. A temperature difference from 4.2 to 7.8 ℃ was observed under working current from 0.01 A to 0.05 A, showing potential application in planar cooling field.
References
[1] E SIMONS R, J EllSWORTH M, C CHU R. An assessment of module cooling enhancement with thermoelectric coolers.. Heat Transf, 127, 76-84(2005).
[2] Y ZHANG H, C MUI Y, M TARIN. Analysis of thermoelectric cooler performance for high power electronic package. Appl. Therm. Eng, 30, 561-568(2010).
[3] C AVRAM B, M IYENGAR, D KRAUS A. Design of optimum plate-fin natural convective heat sinks.. Electronic Packag, 125, 208-216(2003).
[4] E BELL L. Cooling, heating, generating power, and recovering waste heat with thermoelectric systems. Science, 321, 1457-1461(2008).
[5] C HARMAN T, J TAYLOR P, P WALSH M et al. Quantum dot superlattice thermoelectric materials and devices. Science, 297, 2229-2232(2002).
[6] I CHOWDHURY, R PRASHER, K LOFGREE et al. On-chip cooling by superlattice-based thin-film thermoelectrics. Nat. nanotechnol, 4, 235-238(2009).
[7] F HAO, F QIU P, S TANG Y et al. High efficiency Bi2Te3-based materials and devices for thermoelectric power generation between 100 and 300 ℃. Energy & Environ Sci, 9, 3120-3127(2016).
[8] J DISALVO F. Thermoelectric cooling and power generation. Science, 285, 703-706(1999).
[9] J YANG, R STABLER F. Automotive applications of thermoelectric materials.. Electron. Mater, 38, 1245-1251(2009).
[10] Z LU, M LAYANI, X ZHAO et al. Fabrication of flexible thermoelectric thin film devices by inkjet printing. Small, 10, 3551-3554(2014).
[11] B XU, T AGNE M, L FENG T et al. Nanocomposites from solution- synthesized PbTe-BiSbTe nanoheterostructure with unity figure of merit at low-medium temperatures (500-600 K). Adv.Mater, 29(2017).
[12] J ZHU T, P HU L, B ZHAO X et al. New insight into intrinsic point defects in V2VI3 thermoelectric materials. Adv. Sci, 3(2016).
[13] D MADAN, A CHEN, K WRIGHT P et al. Dispenser printed composite thermoelectric thick films for thermoelectric generator application. J. Appl. Phys, 109(2011).
[14] D MADAN, Q WANG Z, A CHEN et al, 104(2014).
[15] J KIM S, H WE J, J CHO B. A wearable thermoelectric generator fabricated on a glass fabric. Energy & Environ Sci, 7, 1959-1965(2014).
[16] T VARGHESE, C HOLLAR, J RICHARDSON et al. High-performance and flexible thermoelectric films by screen printing solution-processed nanoplate crystals. Sci. Rep, 6(2016).
[17] H WU, X LIU, P WEI et al. Fabrication and characterization of brush-printed p-type Bi0.5Sb1.5Te3 thick films for thermoelectric cooling devices.. Electron. Mater, 46, 2950-2957(2016).
[18] X SHI J, L CHEN H, H JIA S et al. Rapid and low-cost fabrication of thermoelectric composite using low-pressure cold pressing and thermocuring methods. Mater. Lett, 212, 299-302(2018).
[19] Z CAO, E KOUKHARENKO, J TUDOR M et al. Flexible screen printed thermoelectric generator with enhanced processes and materials. Sens. Actuators, A, 238, 196-206(2016).
[20] D MADAN, Q WANG Z, A CHEN et al. Enhanced performance of dispenser printed MA n-type Bi2Te3 composite thermoelectric generator. ACS Appl. Mater. Inter, 4, 6117-6124(2012).
[21] D MADAN, Q WANG Z, A CHEN et al. High-performance dispenser printed MA p-type Bi0.5Sb1.5Te3 flexible thermoelectric generators for powering wireless sensor networks. ACS Appl. Mater. Inter, 5, 11872-11876(2013).
[22] K HOU W, L NIE X, Y ZHAO W et al. Fabrication and excellent performance of Bi0.5Sb1.5Te3/epoxy flexible thermoelectric cooling devices. Nano Energy, 50, 766-776(2018).
[23] X GUO, X JIA, J QIN et al. Fast preparation and high thermoelectric performance of the stable Bi0.5Sb1.5Te3 bulk materials for different synthesis pressures. Chem. Phys. Lett, 610, 204-208(2014).
[24] D SUH, S LEE, H MUN et al. Enhanced thermoelectric performance of Bi0.5Sb1.5Te3-expanded grapheme composites by simultaneous modulation of electronic and thermal carrier transport. Nano Energy, 13, 67-76(2015).
[25] D MADAN, Q WANG Z, K WRIGHT P et al. Printed flexible thermoelectric generators for use on low levels of waste heat. Appl.Energy, 156, 587-592(2015).
[26] C CHEN J, J YAN Z, Q WU L. Nonequilibrium thermodynamic analysis of a thermoelectric device. Energy, 22, 979-985(1997).
[27] G CHEN L, C WU, R SUN F. Heat transfer effect on the specific cooling load of refrigerators. Appl. Therm. Eng, 16, 989-997(1996).