
- Chinese Optics Letters
- Vol. 20, Issue 1, 013802 (2022)
Abstract
1. Introduction
Since Birnbaum[
In recent years, most of the works on LIPSS have adopted femtosecond and picosecond laser pulses[
Many parameters such as laser wavelength, pulse duration, fluence, polarization, pulse number, incident angle, and refractive index of environmental media could affect the period size or surface morphology of LIPSS. Nuernberger et al.[
Sign up for Chinese Optics Letters TOC. Get the latest issue of Chinese Optics Letters delivered right to you!Sign up now
2. Experimental Setup
A diagram of the experimental setup is illustrated in Fig. 1, where a
Figure 1.Schematic diagram of the experimental setup.
3. Results and Discussion
Figure 2 shows the SEM images of LIPSS with irradiation fluence ranging from
Figure 2.SEM images of LIPSS with irradiation fluence ranging from 67 mJ/cm2 to 400 mJ/cm2 with the pulse number of 600.
As for the formation of nanoparticles, ablation may be the main reason, as far as we know. Ablation may occur through several different mechanisms, such as phase explosion, evaporation, spallation, and fragmentation[
Figure 3 shows the AFM images of two typical LIPSSs with irradiation fluence of
Figure 3.AFM images of LIPSSs with irradiation fluence of 247 mJ/cm2 and 363 mJ/cm2 under pulse number of 600. (A), (a) 2D AFM image, (B), (b) horizontal height of LIPSS along the green line, and (C), (c) 3D AFM image.
The 2D fast Fourier transform (FFT) and one-dimensional (1D) FFT spectrograms of the SEM images are used to calculate the period of the LIPSS, as shown in Fig. 4. The irradiation fluences are
Figure 4.2D-FFT and 1D-FFT spectrograms of LIPSS SEM images with different fluence: (a), (c) 247 mJ/cm2 and (b), (d) 288 mJ/cm2.
The pulse number applied to the Si surface is another significant parameter that affects the morphology of LIPSS. Figure 5 shows the SEM images of LIPSS with irradiation pulse numbers ranging from 400 to 900 under laser fluence of
Figure 5.SEM images of LIPSS with irradiation pulse number ranging from 400 to 900 under fluence of 247 mJ/cm2.
The incident angle of the laser is the essential parameter to control the period of LIPSS. Based on the theory of interference between the incident laser and surface plasmon polaritons (SPPs), the periodicities dependence of LIPSS on the incident angle of p- and s-polarized light is theoretically predicted by Bauerle[
Figure 6 shows the SEM images of LIPSSs at four different incident angles and the fitting of experimentally obtained periodicities. The periods of LIPSSs at four incident angles are 601 nm, 789 nm, 1147 nm, and 1578 nm, as obtained by 1D-FFT and 2D-FFT. In addition, the period of LIPSS at an incident angle of 10° is about 435 nm. Increasing incident angles is accompanied by growing periodicities. The experiment data is in good agreement with the prediction on the whole.
Figure 6.SEM images of LIPSSs at different incident angles: (a) 20°, (b) 30°, (c) 45°, and (d) 60°. (e) Angular dependence of LIPSS periodicities. Red, blue, and black lines represent, respectively, theoretical values of Λ(p), Λ(s), and fitting of experimental data. Dots represent experimental data of 45° polarization.
Figure 7.SEM images of LIPSS after irradiation with different polarization angles: 0°, 30°, 60°, and 90° under fluence of 207 mJ/cm2 and pulse number of 800. The red arrow indicates the laser polarization direction.
In this work, HWP2 is used to control the polarization angle of the laser. Respectively, the SEM images of LIPSSs after irradiation with different polarization angles of 0°, 30°, 60°, and 90° under laser fluence of
Figure 8.2D-FFT spectrograms of LIPSS in Fig.
After generating LIPSSs on Si, the reflectance spectrum of Si with different morphologies is measured by a spectrophotometer (Hitachi, U-4100). The result is presented in Fig. 9. LIPSS1 is produced with fluence of
Figure 9.Reflectance spectrum of untreated Si and that with two different LIPSSs.
Based on the above discussion of the formation of LIPSS on Si with a nanosecond laser, the relationship between the LIPSS and the laser parameters is shown in Fig. 10. Appropriate fluence and pulse number should be applied to generate LIPSS, because if either of them is too high or too low, it will cause a disappearance of LIPSS. The laser fluence applied to the Si target surface ranges from
Figure 10.General formation of LIPSS at different laser fluence and pulse number with different kinds of surface morphology.
4. Conclusion
In this work, LIPSS was generated on a Si surface by a 532 nm laser with pulse duration of 10 ns with different fluence, pulse number, and incident angle. General formation of LIPSS with different morphology is observed when laser fluence ranges from
References
[1] M. Birnbaum. Semiconductor surface damage produced by ruby lasers. J. Appl. Phys., 36, 3688(1965).
[2] H. Lochbihler. Colored images generated by metallic sub-wavelength gratings. Opt. Express, 17, 12189(2009).
[3] B. Dusser, Z. Sagan, H. Soder, N. Faure, E. Audouard. Controlled nanostructrures formation by ultra fast laser pulses for color marking. Opt. Express, 18, 2913(2010).
[4] J. Long, P. Fan, M. Zhong, H. Zhang, Y. Xie, C. Lin. Superhydrophobic and colorful copper surfaces fabricated by picosecond laser induced periodic nanostructures. Appl. Surf. Sci., 311, 461(2014).
[5] Y. Zhang, G. Zou, L. Liu, Y. Zhao, Q. Liang, A. Wu, Y. N. Zhou. Time-dependent wettability of nano-patterned surfaces fabricated by femtosecond laser with high efficiency. Appl. Surf. Sci., 389, 554(2016).
[6] A. Cunha, A. M. Elie, L. Plawinski, A. P. Serro, A. M. Botelho Do Rego, A. Almeida, M. C. Urdaci, M. C. Durrieu, R. Vilar. Femtosecond laser surface texturing of titanium as a method to reduce the adhesion of Staphylococcus aureus and biofilm formation. Appl. Surf. Sci., 360, 485(2016).
[7] J. Bonse, R. Koter, M. Hartelt, D. Spaltmann, S. Pentzien, S. Hoehm, A. Rosenfeld, J. Krueger. Tribological performance of femtosecond laser-induced periodic surface structures on titanium and a high toughness bearing steel. Appl. Surf. Sci., 336, 21(2015).
[8] G. D. Valle, R. Osellame, P. Laporta. Micromachining of photonic devices by femtosecond laser pulses. J. Opt. A, 11, 013001(2009).
[9] J. Li, S. Ho, M. Haque, P. R. Herman. Nanograting Bragg responses of femtosecond laser written optical waveguides in fused silica glass. Opt. Mater. Express, 2, 1562(2012).
[10] M. Beresna, M. Gecevičius, P. G. Kazansky, T. Taylor, A. V. Kavokin. Exciton mediated self-organization in glass driven by ultrashort light pulses. Appl. Phys. Lett., 101, 053120(2012).
[11] C. W. B. Wu, Z. Luo, J. Li, S. Man, K. Ding, J. Duan. Controllable annulus micro-/nanostructures on copper fabricated by femtosecond laser with spatial doughnut distribution. Chin. Opt. Lett., 18, 013101(2020).
[12] S. G. T. Jiang, Z. Tian, Z. Zhang, L. Niu. Fabrication of diamond ultra-fine structures by femtosecond laser. Chin. Opt. Lett., 18, 101402(2020).
[13] J. Shao, X. Liang, L. You, N. Pan, Y. Lin, S. Wang, Z. Deng, X. Fang, X. Wang. Laser-induced damage and periodic stripe structures of a CaF2 single crystal by an ArF excimer laser. Chin. Opt. Lett., 18, 021403(2020).
[14] L. Gemini, M. Hashida, M. Shimizu, Y. Miyasaka, S. Inoue, S. Tokita, J. Limpouch, T. Mocek, S. Sakabe. Metal-like self-organization of periodic nanostructures on silicon and silicon carbide under femtosecond laser pulses. J. Appl. Phys., 114, 194903(2013).
[15] T. Yang, H. Lin, B. Jia. Ultrafast direct laser writing of 2D materials for multifunctional photonics devices [Invited]. Chin. Opt. Lett., 18, 023601(2020).
[16] Y. Li, J. Hu, W. Liu, J. Yin, J. Lu. High period frequency LIPSS emerging on 304 stainless steel under the irradiation of femtosecond laser double-pulse trains. Chin. Opt. Lett., 19, 123801(2021).
[17] R. Zazo, J. Solis, J. A. Sanchez-Gil, R. Ariza, R. Serna, J. Siegel. Deep UV laser induced periodic surface structures on silicon formed by self-organization of nanoparticles. Appl. Surf. Sci., 520, 146307(2020).
[18] A. G. Cullis, L. T. Canham, P. Calcott. The structural and luminescence properties of porous silicon. J. Appl. Phys., 82, 909(1997).
[19] K. Ahmmed, G. Colin, K. Anne-Marie. Fabrication of micro/nano structures on metals by femtosecond laser micromachining. Micromachines, 5, 1219(2014).
[20] J. Bonse, S. Hohm, S. V. Kirner, A. Rosenfeld, J. Kruger. Laser-induced periodic surface structures—a scientific evergreen. IEEE J. Sel. Top Quantum Electron, 23, 9000615(2017).
[21] A. Y. Vorobyev, C. Guo. Direct femtosecond laser surface nano/microstructuring and its applications. Laser Photonics Rev., 7, 385(2013).
[22] L. Bayera, M. Ehrhardta, P. Lorenza, M. Mäserb, K. Zimmera. Large-area picosecond laser-induced periodic surface structure (LIPSS) on chromium. 9th International Conference on Photonic Technologies LANE, 1(2016).
[23] J. G. A. B. Simoes, R. Riva, W. Miyakawa. High-speed laser-induced periodic surface structures (LIPSS) generation on stainless steel surface using a nanosecond pulsed laser. Surf. Coat. Technol., 344, 423(2018).
[24] M. Sanz, E. Rebollar, R. A. Ganeev, M. Castillejo. Nanosecond laser-induced periodic surface structures on wide band-gap semiconductors. Appl. Surf. Sci., 278, 325(2013).
[25] M. Ehrhardt, B. Han, F. Frost, P. Lorenz, K. Zimmer. Generation of laser-induced periodic surface structures (LIPSS) in fused silica by single NIR nanosecond laser pulse irradiation in confinement. Appl. Surf. Sci., 470, 56(2019).
[26] P. Nuernberger, H. Reinhardt, H. C. Kim, F. Yang, K. Peppler, J. Janek, N. Hampp. Influence of substrate microcrystallinity on the orientation of laser-induced periodic surface structures. J. Appl. Phys., 72, 3688(2015).
[27] S. Camacho-López, R. Evans, L. Escobar-Alarcón, M. A. Camacho-López, M. A. Camacho-López. Polarization-dependent single-beam laser-induced grating-like effects on titanium films. Appl. Surf. Sci., 255, 3028(2008).
[28] A. Y. Vorobyev, C. Guo. Shot-to-shot correlation of residual energy and optical absorptance in femtosecond laser ablation. Appl. Phys. A, 86, 235(2007).
[29] S. Sakabe, M. Hashida, S. Tokita, S. Namba, K. Okamuro. Mechanism for self-formation of periodic grating structures on a metal surface by a femtosecond laser pulse. Phys. Rev. B, 79, 3409(2009).
[30] J. Skolski, G. R. B. E. Roemer, J. V. Obona, A. J. H. I. T. Veld. Modeling laser-induced periodic surface structures: finite-difference time-domain feedback simulations. J. Appl. Phys., 115, 103102(2014).
[31] G. D. Tsibidis, M. Barberoglou, P. A. Loukakos, E. Stratakis, C. Fotakis. Dynamics of ripple formation on silicon surfaces by ultrashort laser pulses in subablation conditions. Phys. Rev. B, 86, 115316(2012).
[32] M. Huang, F. Zhao, Y. Cheng, Z. Xu. Effects of the amorphous layer on laser-induced subwavelength structures on carbon allotropes. Opt. Lett., 37, 677(2012).
[33] T. J.-Y. Derrien, R. Koter, J. Krüger, S. Höhm, A. Rosenfeld, J. Bonse. Plasmonic formation mechanism of periodic 100-nm-structures upon femtosecond laser irradiation of silicon in water. J. Appl. Phys., 116, 074902(2014).
[34] J. Reif, O. Varlamova, S. Varlamov, M. Bestehorn. The role of asymmetric excitation in self-organized nanostructure formation upon femtosecond laser ablation. Appl. Phys. A, 104, 969(2011).
[35] V. I. E. Yanov. Self-organization of ordered nano- and microstructures on the semiconductor surface under the action of laser radiation. Laser Phys., 18, 682(2008).
[36] D. Baeuerle. Laser Processing and Chemistry(2011).
[37] A. M. Bonch-Bruevich, M. N. Libenson, V. S. Makin, V. V. Trubaev. Surface electromagnetic waves in optics. Opt. Eng., 31, 718(1992).
[38] D. E. Aspnes, A. A. Studna. Dielectric functions and optical parameters of Si, Ge, GaP, GaAs, GaSb, InP, InAs, and InSb from 1.5 to 6.0 eV. Phys. Rev. B, 27, 985(1983).
[39] D. R. Austin, K. Kafka, S. Trendafilov, G. Shvets, H. Li, A. Y. Yi, U. B. Szafruga, Z. Wang, Y. H. Lai, C. I. Blaga. Laser induced periodic surface structure formation in germanium by strong field mid IR laser solid interaction at oblique incidence. Opt. Express, 23, 19522(2015).
[40] F. Garrelie, J. P. Colombier, F. Pigeon, S. Tonchev, N. Faure, M. Bounhalli, S. Reynaud, O. Parriaux. Evidence of surface plasmon resonance in ultrafast laser-induced ripples. Opt. Express, 19, 9035(2011).
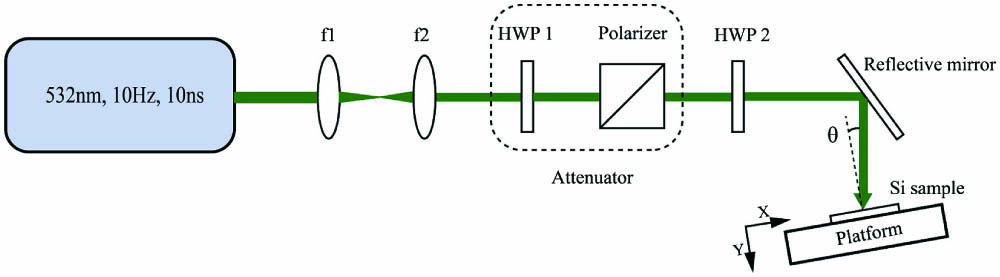
Set citation alerts for the article
Please enter your email address