
- Journal of Semiconductors
- Vol. 40, Issue 8, 081505 (2019)
Abstract
1. Introduction
It is an everlasting dream to make electronic devices miniaturization, multi-functionalization, intellectualization, and low-power consumption in this current information society[
DMSs researches date back to the concentrated magnetic semiconductors compounds, like EuX[
It is of great fundamental significance and practical value to further improve TC of (Ga,Mn)As[
Figure 1.(Color online) The crystal structure of (a) “111” Li(Zn,Mn)As with zinc blende structure, (b) “122” (Ba,K)(Zn,Mn)2As2 with ThCr2Si2 type structure, (c) (Sr,Na)(Zn,Mn)2As2 with CaAl2Si2 type structure, (d) “1111” (Ba,K)F(Zn,Mn)As with ZrCuSiAs structure. Adoped from Refs. [
2. Polycrystals synthesis and basic physicial properties
2.1. “111” type DMSs
LiZnAs[
Figure 2.(Color online) Magnetization & transport measurements of Li(Zn,Mn)As. (a) The temperature dependence of
In order to examine volume fraction and the ordered moment size, μSR measurements were performed on sintered polycrystalline specimens of Li1.1(Zn0.95Mn0.05)As[
Figure 3.(Color online) Results of
The discovery of Li(Zn,Mn)As sparked extensive researches in this 111 type DMSs[
Figure 4.(Color online) (a)
Usually, the carriers are induced in Li site while spins in Zn site in all these above systems. Different from that idea, a new DMSs Li(Zn,Co,Mn)As[
2.2. “122” type DMSs
BaZn2As2 is a semiconductor synthetized at high temperature (>900 °C) with the tetragonal ThCr2Si2 crystal structure. A new type “122” DMSs (Ba,K)(Zn,Mn)2As2 has been synthesized with the Ba2+/K1+ substitution (hole carries) and Zn2+/Mn2+ (spins) doping. Fig. 5(a) shows the temperature dependence of M in H = 500 Oe for (Ba0.7K0.3)(Zn0.85Mn0.15)2As2 at ZFC and FC procedures with TC 230 K. The hysteresis curves M(H) are shown in the inset of Fig. 5(a). Fig. 5(b) exhibits the spontaneous magnetization curve under 5 Oe of (Ba0.7K0.3)- (Zn0.85Mn0.15)2As2, showing T3/2 dependence in low temperature, as expected for a homogeneous ferromagnet[
Figure 5.(Color online) Magnetization & transport measuremets of (Ba,K)(Zn,Mn) 2As2. (a) The tempertature dependence of
Different from (Ba,K)(Zn,Mn)2As2, another “122” type DMSs with hexagonal CaAl2Si2 was reported subsequently, such as (Ca,Na)(Zn,Mn)2As2[
Figure 6.(Color online) Magnetization & transport measurements of (Ca,Na)(Zn,Mn) 2As2, (Sr,Na)(Zn,Mn)2As2 and (Sr,Na)(Cd,Mn)2As2. (a) The temperature dependence of
2.3. “1111” type DMSs
Isostructural to the well-studied iron-based superconductor LaFeAs(O1–xFx)[
Figure 7.(Color online) (a) Field dependences of magnetization for (La0.95Ca0.05)(Zn0.9Mn0.1)SbO measured at 25 and 100 K. (b) resistivity of (La0.95Ca0.05)(Zn0.925Mn0.075)SbO in various external field
3. Properties of (Ba,K)(Zn,Mn)2As2
3.1. X ray absorption spectroscopy (XAS) & angle-resolved photoemission spectroscopy (ARPES)
The origin of magnetic ordering on DMSs is still full of debates[
Fig. 8(a) exhibits the X-ray absorption spectroscopy (XAS) measurements[
Figure 8.(Color online) (a) Mn
3.2. Single crystal growth and spin polarization measurements
Compared with polycrystals, single crystals are ideal research platforms due to fewer defects. (Ba,K)(Zn,Mn)2As2 single crystal[
Figure 9.(Color online) (a) The X-ray difraction patterns of (Ba0.904K0.096)(Zn0.805Mn0.195)2As2 at room temperature. The inset shows the crystal structure (right) and its photograph (lef). (b) The tempertature dependence of
Andreev refection spectroscopy (AR spectroscopy) is commonly used to achieve spin polarization (P) in various materials, e.g., (Ga,Mn)As[
Figure 10.(Color online) (a) Sketch of the (Ba0.904K0.096)(Zn0.805Mn0.195)2As2/Pb junctions used for Andreev refection spectroscopy. The inset is the normalization for the diferential conductance
Compared to classical DMSs, new type of DMSs has one great advantage, existence of numerous isostructual function materials. As shown in Fig. 11, (Ba,K)(Zn,Mn)2As2 shares the same tetragonal ThCr2Si2 type structure with semiconductor BaZn2As2, antiferromagnetic BaMn2As2[
Figure 11.(colour online) Crystal structures and key physical propertity of (Ba,K)(Zn,Mn)2As2, BaMn2As2, BaZn2As2 and (Ba,K)Fe2As2.
4. Summary and outlook
There are three main group of new diluted magnetic semiconductors with independent charge and spin doping, i.e. the “111”, “122” and “1111” type, as listed in Table 1. BZA is unique in that it has TC above 200 K. Note that there are other four DMSs which are isostructural to BZA but have low TC. What makes BZA so special is one open question. The accurate answer to the question could be the clue to seek mechanism of DMSs and the guiding light to search for room temperature ferromagnetic DMSs.
Taking the advantage of lattice matched superconductors, semiconductors and antiferromagnetic compounds, these new types of DMSs have potential to fabricate multilayer heterojunctions[
Acknowledgment
We would like to thank the collaborators Prof. Y. J. Uemura, Prof. Yong Qing Li, Prof. A. Fujimori, Prof. S. Maekawa, Prof. Bo Gu, Prof. Lixin Cao, et al. We are grateful to Prof. L. Yu, F. C. Zhang, J. H. Zhao and F. L. Ning for the helpful discussions. This work is financially supported by Ministry of Science and Technology of China (Nos. 2018YFA03057001, and 2017YFB0405703), National Natural Science Foundation of China through the research projects (No. 11534016).
References
[1]
[2] C A Mack. Fifty years of Moore's law. IEEE Trans Semicond Manufac, 24, 202(2011).
[3] I Žutić, J Fabian, Sarma S Das. Spintronics fundamentals and applications. Rev Mod Phys, 76, 323(2004).
[4] I Žutić, T Zhou. Tailoring magnetism in semiconductors. Sci Chin Phys, Mechan Astronom, 61, 067031(2018).
[5] T R McGuire, e B E Argyle, r M W Shafer et al. Magnetic properties of some divalent europium compounds. J Appl Phys, 34, 1345(1963).
[6] S B Berger, h H L Pinch. Ferromagnetic resonance of single crystals of CdCr2S4 and CdCr2Se4. J Appl Phys, 38, 949(1967).
[7] J K Furdyna. Diluted magnetic semiconductors. J Appl Phys, 64, R29(1988).
[8] T Story, a R R Galazka, l R B Frankel et al. Carrier-concentration-induced ferromagnetism in PbSnMnTe. Phys Rev Lett, 56, 777(1986).
[9] N Samarth, a J K Furdyna. Diluted magnetic semiconductors. Proc IEEE, 78, 990(1990).
[10] H Munekata, H Ohno, S von Molnar. Diluted magnetic III–V semiconductors. Phys Rev Lett, 63, 1849(1989).
[11] H Ohno, n A Shen, a F Matsukura et al. (Ga,Mn)As: A new diluted magnetic semiconductor based on GaAs. Appl Phys Lett, 69, 363(1996).
[12] H Ohno. Making nonmagnetic semiconductors ferromagnetic. Science, 281, 951(1998).
[13] H Ohno, H Munekata, y T Penney et al. Magnetotransport properties of p-type (In,Mn)As diluted magnetic III–V semiconductors. Phys Rev Lett, 68, 2664(1992).
[14] M Wang, n R P Campion, h A W Rushforth et al. Achieving high Curie temperature in (Ga,Mn)As. Appl Phys Lett, 93, 132103(2008).
[15] L Chen, n S Yan, u P F Xu et al. Low-temperature magnetotransport behaviors of heavily Mn-doped (Ga,Mn)As films with high ferromagnetic transition temperature. Appl Phys Lett, 95, 182505(2009).
[16] L Chen, g X Yang, g F Yang et al. Enhancing the Curie temperature of ferromagnetic semiconductor (Ga,Mn)As to 200 K via nanostructure engineering. Nano Lett, 11, 2584(2011).
[17] T Dietl. A ten-year perspective on dilute magnetic semiconductors and oxides. Nat Mater, 9, 965(2010).
[18] A Bonanni, l T Dietl. A story of high-temperature ferromagnetism in semiconductors. Chem Soc Rev, 39, 528(2010).
[19] Z Deng, n C Q Jin, u Q Q Liu et al. Li(Zn,Mn)As as a new generation ferromagnet based on a I–II–V semiconductor. Nat Commun, 2, 422(2011).
[20] K Zhao, g Z Deng, g X C Wang et al. New diluted ferromagnetic semiconductor with Curie temperature up to 180 K and isostructural to the '122' iron-based superconductors. Nat Commun, 4, 1442(2013).
[21] Z Deng, o K Zhao, n C Jin. New types of diluted magnetic semiconductors with decoupled charge and spin doping. Physics, 42, 682(2013).
[22] K Zhao, B Chen, o G Zhao et al. Ferromagnetism at 230 K in (Ba0.7K0.3)(Zn0.85Mn0.15)2As2 diluted magnetic semiconductor. Chin Sci Bull, 59, 2524(2014).
[23] R Bacewicz, k T F Ciszek. Preparation and characterization of some AIBIICV type semiconductors. Appl Phys Lett, 52, 1150(1988).
[24] K Kuriyama, a F Nakamura. Electrical transport properties and crystal structure of LiZnAs. Phys Rev B, 36, 4439(1987).
[25] K Kuriyama, T Kato, a K Kawada. Optical band gap of the filled tetrahedral semiconductor LiZnAs. Phys Rev B, 49, 11452(1994).
[26] X C Wang, u Q Q Liu, v Y X Lv et al. The superconductivity at 18 K in LiFeAs system. Solid State Commun, 148, 538(2008).
[27] J Masek, y J Kudrnovsky, F Maca et al. Dilute moment n-type ferromagnetic semiconductor Li(Zn,Mn)As. Phys Rev Lett, 98, 067202(2007).
[28] Z Deng, o K Zhao, u B Gu et al. Diluted ferromagnetic semiconductor Li(Zn,Mn)P with decoupled charge and spin doping. Phys Rev B, 88, 081203(2013).
[29] Y J Uemura, i T Yamazaki, n D R Harshman et al. Muon-spin relaxation in AuFe and CuMn spin glasses. Phys Rev B, 31, 546(1985).
[30] S R Dunsiger, o J P Carlo, o T Goko et al. Spatially homogeneous ferromagnetism of (Ga,Mn)As. Nat Mater, 9, 299(2010).
[31] Y J Uemura, T Goko, I M Gat-Malureanu et al. Phase separation and suppression of critical dynamics at quantum phase transitions of MnSi and (Sr1–
[32] F L Ning, n H Man, g X Gong et al. Suppression of
[33] B Chen, g Z Deng, i W Li et al. Li(Zn,Co,Mn)As: A bulk form diluted magnetic semiconductor with Co and Mn co-doping at Zn sites. AIP Adv, 6, 115014(2016).
[34] S L Guo, o Y Zhao, H Y Man et al.
[35] F Sun, u C Xu, u S Yu et al. Synchrotron X-ray diffraction studies on the new generation ferromagnetic semiconductor Li(Zn,Mn)As under high pressure. Chin Phys Lett, 34, 067501(2017).
[36] W Han, n B J Chen, u B Gu et al. Li(Cd,Mn)P: a new cadmium based diluted ferromagnetic semiconductor with independent spin & charge doping. Sci Rep, 9, 7490(2019).
[37] F Matsukura, i M Sawicki, l T Dietl et al. Magnetotransport properties of metallic (Ga,Mn)As films with compressive and tensile strain. Physica E, 21, 1032(2004).
[38] A H MacDonald, r P Schiffer, h N Samarth. Ferromagnetic semiconductors: moving beyond (Ga,Mn)As. Nat Mater, 4, 195(2005).
[39] T Sasaki, a S Sonoda, o Y Yamamoto et al. Magnetic and transport characteristics on high Curie temperature ferromagnet of Mn-doped GaN. J Appl Phys, 91, 7911(2002).
[40] K Zhao, n B J Chen, g Z Deng et al. (Ca,Na)(Zn,Mn)2As2: A new spin and charge doping decoupled diluted ferromagnetic semiconductor. J Appl Phys, 116, 163906(2014).
[41] B Chen, g Z Deng, i W Li et al. (Sr1–
[42] B J Chen, o K Zhao, Z Deng et al. (Sr,Na)(Zn,Mn)2As2: A diluted ferromagnetic semiconductor with the hexagonal CaAl2Si2 type structure. Phys Rev B, 90, 155202(2014).
[43] T Dietl. Interplay between carrier localization and magnetism in diluted magnetic and ferromagnetic semiconductors. J Phys Soc Jpn, 77, 031005(2008).
[44] Y Kamihara, e T Watanabe, o M Hirano et al. Iron-based layered superconductor La(O1–
[45] W Han, o K Zhao, g X Wang et al. Diluted ferromagnetic semiconductor (LaCa)(ZnMn)SbO isostructural to " 1111” type iron pnictide superconductors. Sci Chin Phys, Mechan Astronom, 56, 2026(2013).
[46] B J Chen, g Z Deng, g X C Wang et al. Structural stability at high pressure, electronic, and magnetic properties of BaFZnAs: A new candidate of host material of diluted magnetic semiconductors. Chin Phys B, 25, 077503(2016).
[47] B Chen, g Z Deng, W Li et al. New fluoride-arsenide diluted magneticsemiconductor (Ba,K)F(Zn,Mn)As with independent spinand charge doping. Sci Rep, 6, 36578(2016).
[48]
[49] T Jungwirth, J Wunderlich, Novák V V et al. Spin-dependent phenomena and device concepts explored in (Ga,Mn)As. Rev Mod Phys, 86, 855(2014).
[50] H Ohno, a D Chiba, a F Matsukura et al. Electric-field control of ferromagnetism. Nature, 408, 944(2000).
[51] T Dietl, o H Ohno, a F Matsukura et al. Zener model description of ferromagnetism in zinc-blende magnetic semiconductors. Science, 287, 1019(2000).
[52] T Dietl, o H Ohno, F Matsukura. Hole-mediated ferromagnetism in tetrahedrally coordinated semiconductors. Phys Rev B, 63, 195205(2001).
[53] T Jungwirth, a J Sinova, k J Masek et al. Theory of ferromagnetic (III,Mn)V semiconductors. Rev Mod Phys, 78, 809(2006).
[54] K Sato, L Bergqvist, J Kudrnovský et al. First-principles theory of dilute magnetic semiconductors. Rev Mod Phys, 82, 1633(2010).
[55] T Dietl, o K Sato, a T Fukushima et al. Spinodal nanodecomposition in semiconductors doped with transition metals. Rev Modern Phys, 87, 1311(2015).
[56] T Dietl, o H Ohno. Dilute ferromagnetic semiconductors: Physics and spintronic structures. Rev Mod Phys, 86, 187(2014).
[57] D J Keavney, u D Wu, d J W Freeland et al. Element resolved spin configuration in ferromagnetic manganese-doped gallium arsenide. Phys Rev Lett, 91, 187203(2003).
[58] B Beschoten, l P Crowell, h I Malajovich et al. Magnetic circular dichroism studies of carrier-induced ferromagnetism in (Ga1−
[59] J K Glasbrenner, I Žutić, I I Mazin. Theory of Mn-doped II–II–V semiconductors. Phys Rev B, 90, 140403(2014).
[60] H Suzuki, o K Zhao, a G Shibata et al. Photoemission and x-ray absorption studies of the isostructural to Fe-based superconductors diluted magnetic semiconductor Ba1−
[61] Y Takeda, i M Kobayashi, e T Okane et al. Nature of magnetic coupling between Mn ions in As-grown Ga1–
[62] J I Hwang, i M Kobayashi, g G S Song et al. X-ray magnetic circular dichroism characterization of GaN∕Ga1−xMnxN digital ferromagnetic heterostructure. Appl Phys Lett, 91(2007).
[63] S Andrieu, y E Foy, r H Fischer et al. Effect of O contamination on magnetic properties of ultrathin Mn films grown on (001) Fe. Phys Rev B, 58, 8210(1998).
[64] H Suzuki, a T Yoshida, a S Ideta et al. Absence of superconductivity in the hole-doped Fe pnictide Ba(Fe1−
[65] T Burnus, u Z Hu, h H H Hsieh et al. Local electronic structure and magnetic properties of LaMn0.5Co0.5O3 studied by x-ray absorption and magnetic circular dichroism spectroscopy. Phys Rev B, 77, 125124(2008).
[66] H Suzuki, o G Q Zhao, o K Zhao et al. Fermi surfaces and p−d hybridization in the diluted magnetic semiconductor Ba1−
[67] G Q Zhao, n C Q Lin, g Z Deng et al. Single crystal growth and spin polarization measurements of diluted magnetic semiconductor (BaK)(ZnMn)2As2. Sci Rep, 7, 14473(2017).
[68] G Q Zhao, i Z Li, n F Sun et al. Effects of high pressure on the ferromagnetism and in-plane electrical transport of (Ba0.904K0.096)(Zn0.805Mn0.195)2As2 single crystal. J Phys Condens Matter, 30, 254001(2018).
[69] N Nagaosa, a J Sinova, a S Onoda et al. Anomalous Hall effect. Rev Mod Phys, 82, 1539(2010).
[70] J G Braden, r J S Parker, g P Xiong et al. Direct measurement of the spin polarization of the magnetic semiconductor (Ga,Mn)As. Phys Rev Lett, 91, 056602(2003).
[71] R P Panguluri, y B Nadgorny, z T Wojtowicz et al. Inelastic scattering and spin polarization in dilute magnetic semiconductor (Ga,Mn)Sb. Appl Phys Lett, 91, 252502(2007).
[72] M Bowen, M Bibes, A Barthélémy et al. Nearly total spin polarization in La2/3Sr1/3MnO3 from tunneling experiments. Appl Phys Lett, 82, 233(2003).
[73] J M D Coey, o S Sanvito. Magnetic semiconductors and half-metals. J Phys D, 37, 988(2004).
[74] C Ren, c J Trbovic, r R L Kallaher et al. Measurement of the spin polarization of the magnetic semiconductor EuS with zero-field and Zeeman-split Andreev reflection spectroscopy. Phys Rev B, 75, 205208(2007).
[75] T Guan, n C Lin, g C Yang et al. Evidence for half-metallicity in n-type HgCr2Se4. Phys Rev Lett, 115, 087002(2015).
[76] G E Blonder, m M Tinkham, k T M Klapwijk. Transition from metallic to tunneling regimes in superconducting microconstrictions: Excess current, charge imbalance, and supercurrent conversion. Phys Rev B, 25, 4515(1982).
[77] G Gu, o G Zhao, n C Lin et al. Asperomagnetic order in diluted magnetic semiconductor (Ba,Na)(Zn,Mn)2As2. Appl Phys Lett, 112, 032402(2018).
[78] Y Singh, n M A Green, g Q Huang et al. Magnetic order inBaMn2As2 from neutron diffraction measurements. Phys Rev B, 80, 100403(2009).
[79] M Rotter, l M Tegel, D Johrendt. Superconductivity at 38 K in the iron arsenide (Ba1–
[80] S Guo, n H Man, g K Wang et al. Ba(Zn,Co)2As2: A diluted ferromagnetic semiconductor with n-type carriers and isostructural to 122 iron-based superconductors. Phys Rev B, 99, 155201(2019).
[81] A Hirohata, a H Sukegawa, H Yanagihara et al. Roadmap for emerging materials for spintronic device applications. IEEE Trans Magnet, 51, 0800511(2015).
[82] A Beleanu, s J Kiss, r G Kreiner et al. Large resistivity change and phase transition in the antiferromagnetic semiconductors LiMnAs and LaOMnAs. Phys Rev B, 88, 184429(2013).
[83] Y Peng, u S Yu, o G Q Zhao et al. Effects of chemical pressure on diluted magnetic semiconductor (Ba,K)(Zn,Mn)2As2. Chin Phys B, 28, 057501(2019).
[84] B A Frandsen, g Z Gong, n M W Terban et al. Local atomic and magnetic structure of dilute magnetic semiconductor (Ba,K)(Zn,Mn)2As2. Phys Rev B, 94, 094102(2016).
[85] F Sun, i N N Li, n B J Chen et al. Pressure effect on the magnetism of the diluted magnetic semiconductor (Ba1−
[86] F Sun, o G Q Zhao, a C A Escanhoela et al. Hole doping and pressure effects on the II–II–V-based diluted magnetic semiconductor (Ba1−
[87] M A Surmach, B J Chen et al. Weak doping dependence of the antiferromagnetic coupling between nearest-neighbor Mn2+ spins in (Ba1–
[88] R Wang, g Z X Huang, o G Q Zhao et al. Out-of-plane easy-axis in thin films of diluted magnetic semiconductor Ba1−
[89] H C Yang, u K Liu, u Z Y Lu. Magnetic interactions in a proposed diluted magnetic semiconductor (Ba1–
[90] B Gu, a S Maekawa. Diluted magnetic semiconductors with narrow band gaps. Phys Rev B, 94, 155202(2016).
[91] H Man, o S Guo, i Y Sui et al. Ba(Zn(1–2
[92] S Guo, n H Man, g X Gong et al. (Ba1–
[93] X Yang, n Q Chen, Y Li et al. Sr0.9K0.1Zn1.8Mn0.2As2: A ferromagnetic semiconductor with colossal magnetoresistance. EPL, 107, 67007(2014).
[94] J T Yang, o S J Luo, g Y C Xiong. Magnetic mechanism investigations on K and Mn co-doped diluted magnetic semiconductor (Sr,K)(Zn,Mn)2As2. J Magnet Magnet Mater, 407, 334(2016).
[95] X Yang, i Y Li, g P Zhang et al. K and Mn co-doped BaCd2As2: A hexagonal structured bulk diluted magnetic semiconductor with large magnetoresistance. J Appl Phys, 114, 223905(2013).
[96] N Emery, n E J Wildman, e J M S Skakle et al. Variable temperature study of the crystal and magnetic structures of the giant magnetoresistant materials LMnAsO (L = La, Nd). Phys Rev B, 83, 144429(2011).
[97] C Ding, o S Guo, o Y Zhao et al. The synthesis and characterization of 1111 type diluted ferromagnetic semiconductor (La1–
[98] C Jin, g X Wang, u Q Liu et al. New quantum matters: Build up versus high pressure tuning. Sci Chin Phy, Mechan Astronom, 56, 2337(2013).
[99] C Ding, n H Man, n C Qin et al. (La1–
[100] X Li, u X Wu, g J Yang. Control of spin in a La(Mn,Zn)AsO alloy by carrier doping. J Mater Chem C, 1, 7197(2013).
[101] X Yang, i Y Li, n C Shen et al. Sr and Mn co-doped LaCuSO: A wide band gap oxide diluted magnetic semiconductor with
[102] J Lu, n H Man, g C Ding et al. The synthesis and characterization of 1111-type diluted magnetic semiconductors (La1–
[103] Y Zhao, g K Wang, o S Guo et al. La(Zn 1–2
[104] S Guo, o Y Zhao, g X Gong et al. La(Zn1−2
[105] L Fu, u Y Gu, o S Guo et al. Ferromagnetism in fluoride-antimonide SrF(Zn1–2
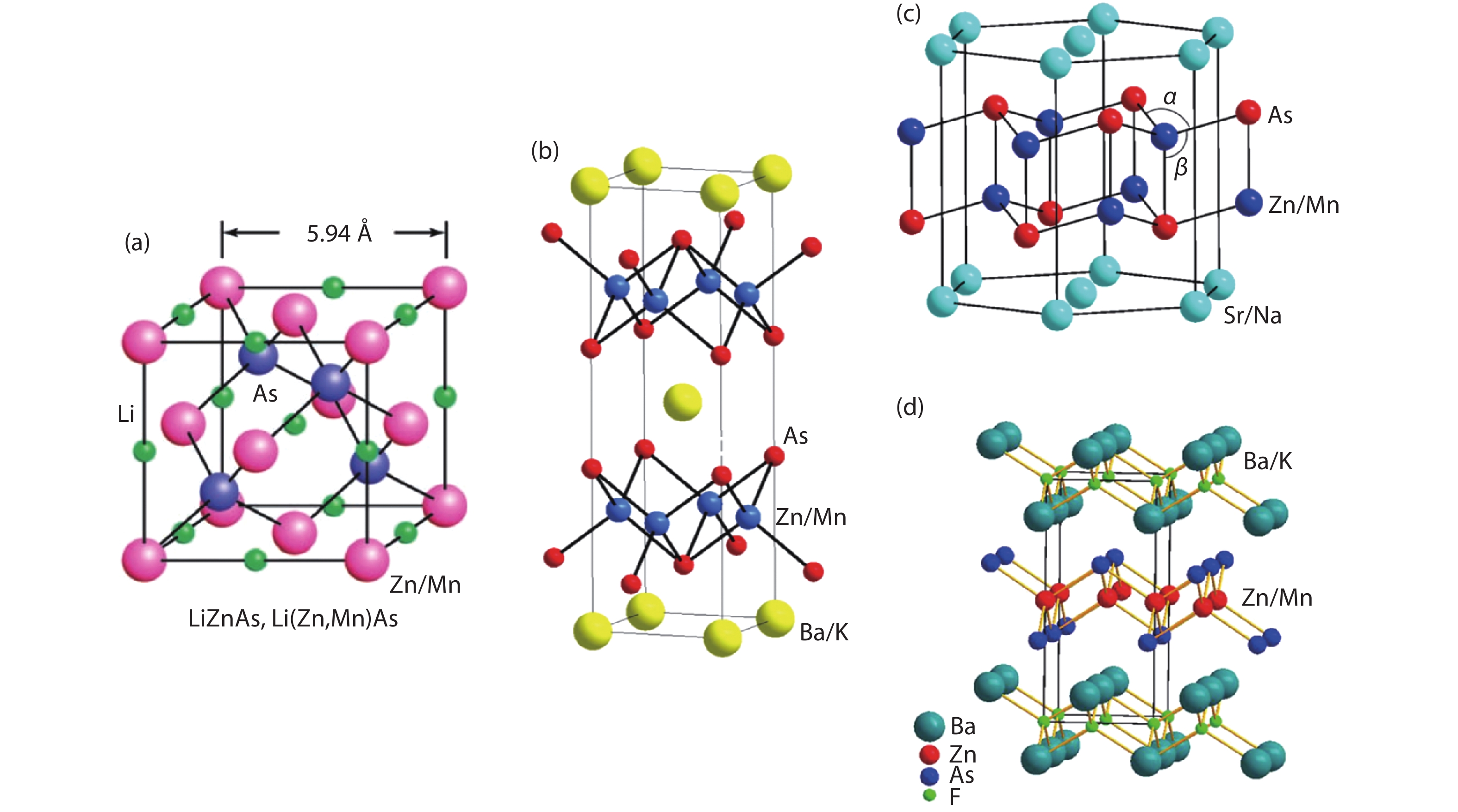
Set citation alerts for the article
Please enter your email address