
- Journal of Semiconductors
- Vol. 41, Issue 1, 011701 (2020)
Abstract
1. Introduction
Nowadays, the fast-growing energy consumption and increasingly serious environmental pollution are two of the most important issues in the world, so the development of renewable and clean energies is an urgent problem to be solved. As a clean energy, hydrogen gas (H2) is one of the most promising energy carriers to substitute traditional fossil fuels for solving the environmental issues, which is attribute to that H2 has many excellent properties such as twice more gravimetric energy density (120 versus 44 MJ/kg) than that of traditional fossil fuels, transportable and storable nature, renewable energy with zero-carbon emission etc[
Among a variety of solar water splitting technologies, the development and design of semiconductor based photocatalytic or photoelectrocatalytic solar water splitting systems have attracted considerable and growing attention in the past few decades[
In this paper, the recent developments of perovskite photocatalysts for solar water splitting are comprehensively reviewed. It will focus on the designing and modulating of different perovskite materials to enhance their light absorption capability and the photocatalytic activity for water splitting. The aim of this review is to summarize recent findings and progress on perovskite based photocatalysts and provide some useful guidance for the future research on the design and development of highly efficient perovskite based photocatalysts and systems for solar water splitting. Based on this, the paper is composed of five sections. Section 1 briefly introduces the relationship between hydrogen energy and the crisis of energy and environment in the world and the perovskite materials. In Section 2, the structures and properties of perovskite materials are presented. In Section 3 the fundamentals of solar water splitting including photocatalysis and photoelectrocatalysis are discussed. In Section 4, the recent developments and strategies regarding the design of perovskite catalysts for photocatalytic reactions, including modification of the chemical component, crystal structure and morphology engineering, application of ferroelectric property, construction of heterojunction structure, and construction of plasmonic-based photocatalysts, are comprehensively reviewed. Finally, in Section 5, the current challenges and perspectives for the future research in developing of perovskite based photocatalysts for water splitting are discussed.
2. Structures and properties of perovskite materials
Perovskite materials generally have a same crystal structure as calcium titanate (CaTiO3), which is initially discovered by the Russian mineralogist Lev Perovski. Later, the “perovskite” is used to represent for a family of materials that have the same type of crystalline unit cell as CaTiO3[
Figure 1.(Color online) (a) Schematic structure of cubic ABX3 perovskite unit cell, and (b) the extended tridimensional structure of perovskites formed by the corner-linked octahedral.
As for the perovskite materials, the cations occupying in the A and B site can affect the properties of the materials. More than 90% of metal elements in the periodic table can be stably located in the perovskite lattice, and many kinds of compositions and constituent elements essentially can not change the basic structure. In the ideal cubic symmetry structure, the ionic radii keep the following relationship: rA + rX =
The rich compositions and constituent elements of perovskite materials gives rise to the great flexiblility of their electronic structures and band structures, which in turn makes the perovskites a wide variety of diverse physical and chemical properties, such as fine ferroelectricity, superconductivity, colossal magneto resistance, perfect oxygen ion diffusion property, lithium mobility and high catalytic performance (including oxidation of small molecules such as CO and hydrocarbons, photo(electro)chemical splitting of water, and reduction of CO2 and O2[
3. Fundamentals of solar water splitting
As a potential candidate for the storage and conversion of solar energy, the photo(electro)catalytic water splitting into hydrogen and oxygen has been regarded as one of the most important methods for the future energy crisis. Since the solar water splitting was firstly discovered by Honda and Fujishima in 1972, which showed that the solar water splitting can be occurred when using single-crystalline TiO2 as a photoanode under UV light irradiation and Pt as a cathode with an external bias[
Figure 2.(Color online) Schematic illustration of the basic reaction mechanism of the overall water splitting on a semiconductor (a), and uphill reaction process of photocatalytic water splitting (b). Reprinted from Ref. [
As shown in Fig. 2(b), the solar water-splitting is an uphill and nonspontaneous reaction because the Gibbs free energy is positive change about 237.3 kJ/mol under standard temperature and pressure[
There are mainly two ways for solar water splitting: the one is using powdered photocatalyst for water splitting and another is PEC method that using photoelectrodes. The basic feature of photocatalytic water splitting using a powdered photocatalyst is shown in Fig. 2(b). The pulverized photocatalyst is well dispersed in a water pool and exposed to sunlight, and then the generated gas from water splitting can be readily got. For the simplicity of powdered photocatalyst system, it is advantageous for large-scale application. However, the dependence on using sacrificial agent for the half-reaction and the difficulty for separation hydrogen and oxygen from the resulting gas mixture are the main challenge of this method. Thus, it is important to develop efficient photocatalyst and design optimized system for practical application of solar water splitting by powdered photocatalyst. In addition, lots of PEC cells have also been developed for solar water splitting[
Figure 3.(Color online) (a) Schematic illustration of water splitting using a TiO2 photoelectrode. Reprinted from Ref. [
According to the previous researches[
Figure 4.(Color online) Schematic illustration of the main processes of the photocatalytic overall water splitting reaction.
4. Strategies to apply perovskite materials for solar water splitting
To design a photocatalyst with excellent performance, the generation, migration and reaction of the photogenerated charge carriers, which are three major steps during the whole process of photocatalytic reaction, must be considered. Perovskite can be used as a new category of ideal photocatalyst for solar water splitting because it has many advantageous properties different from other conventional photocatalytic materials. Firstly, some of perovskites have suitable band structure that are favorable for either half reaction or the overall water splitting. Secondly, the flexible compositions and constituent elements of perovskite materials in A site or B site offer its adjustable electronic structure and the according band structure. Last but not least, some of perovskites have ferroelectric and/or piezoelectric properties which can further enhance their photocatalytic abilities for water splitting[
4.1. Modification of the chemical component
As the electronic band structures of perovskite materials are directly related to their chemical component, the strategy to modify the chemical component is mainly for the purpose of adjusting the electronic structures and enhancing the solar light response of the photocatalytic materials. The electronic band structures of a perovskite photocatalyst can be designed and modified by doping of foreign element. Generally, the CB of most perovskite materials is mainly consisted of d0 transition metal orbits and the bottom edge of CB is much lower than the redox potential of H+/H2 (0 V vs. NHE). The VB is mainly consisted of O2p atomic obits and the top edge of the VB energy levels is much higher than the redox potential of H2O/O2. The large band gap can be altered in two main ways: introducing new mid-gap state between the VB and CB through modifying the chemical component of perovskite material, and altering the VB or CB position of perovskite material through the mixing of energy levels of the foreign elements and host elements[
In the past few years, numerous doped perovskite photocatalysts have been explored for potential applications in the field of photocatalysis, and the most common materials for solar water splitting under UV and visible lights are Ti, Ta, and Nb-based perovskite oxides[
Figure 5.(Color online) Schematic illustration of (a) the band structure and (b) DOS (density of states) plots of LCTO, (c) typical time course of photocatalytic H2 evolution reaction, (d) photocatalytic O2 evolution rate as a function of the cutoff wavelength of incident light. Reprinted from Ref. [
In addition to one element or one site doped of perovskite materials, many two elements doped perovskite photocatalysts with strong visible light absorption have been reported. For example, Cr, B-codoped-SrTiO3 had been synthesized by Yu et al. The narrowed band gap of this material with strong visible light absorption is due to strong p-d repulsion of doped B2p orbits with Cr3dorbits. The results offer a novel route that co-doping of two elements can also modify the band structure of perovskite materials[
Some researchers have also investigated the solid solutions that are composed of two different perovskite materials to overcome the limitations of wide band gap for solar water splitting, including Na0.5La0.5TiO3-LaCrO3, AgNbO3-SrTiO3, SrTiO3-LaTiO2N, and BiFeO3-SrTiO3 (BFO-STO)[
Figure 6.(Color online) (a) Schematic diagram of band positions relative to the vacuum level and NHE for the BFO50-STO50 film. A, B, and C represent the photoexcited carriers relaxation and recombination processes. (b) Current density-potential curves of BFO, BFO75-STO25, BFO50-STO50, BFO25-STO75, and STO films. Reprinted from Ref. [
4.2. Crystal structure and morphology engineering
In general, the crystal structure, crystallinity, particle shape and size of the photocatalyst are directly related to the surface area of photocatalysts and the diffusion pathway of charge carriers, which can directly affect the photocatalytic activity of the photocatalysts[
Figure 7.(Color online) The morphology engineering strategy for SrTiO3 nanocrystals. (a–c) The morphology of 6-facet SrTiO3 nanocrystals. (d) The structure of the {001} facet of SrTiO3. (e) The schematic description of changing SrTiO3 nanocrystals from 6-facet to 18-facet. (f–h) The morphology of 18-facet SrTiO3 nanocrystals. (i) The structure of the {110} facet of SrTiO3. Reprinted from Ref. [
Some researchers have also investigated the effects of restructuring the crystal structure of the perovskite on the electronic structure and the according photocatalytic performance of photocatalyst for solar water splitting. By means of systematic density functional theory (DFT) computations, Qiao et al. introduced a two-dimensional photocatalyst of monolayer PdSeO3, which has a moderate band gap of 2.84 eV and has rather pronounced optical absorption both in the visible and ultraviolet regions of the solar spectrum. Remarkably, the valence and conduction bands of PdSeO3 perfectly engulf the redox potentials of water, which make it can be utilized as a highly efficient photocatalyst for direct overall water splitting without using sacrificial reagents and cocatalysts[
Figure 8.(Color online) (a) UV–vis absorption spectra and (b) typical time course of photocatalytic H2 evolution reaction of c-NaNbO3 (cubic NaNbO3) and o-NaNbO3 (orthorhombic NaNbO3) samples. Reprinted from Ref. [
Particle size can also influence the photocatalytic activity. Since the smaller particles have a larger specific surface area and smaller period for migration of the photogenerated carriers to the surface of photocatalysts, which could reduce the probability of charge recombination and improve the photocatalytic activity of photocatalysts. Parida et al. synthesized LaFeO3 NPs with different particle sizes by a sol-gel method under different activated temperatures from 500 to 900 °C for 2 h[
4.3. Application of ferroelectric property
Due to their unique performances in the separation and migration photoexcited charge carriers, the application of ferroelectric materials for solar water splitting is another potentially efficient approach to overcome the limits of the traditional semiconductors[
Figure 9.(Color online) (a) External quantum yield spectra measured for BFO electrodes before poling and after + 8 and –8 V poling. (b) Photocurrent-potential characteristics of the photoelectrodes with different polarization states. Schematic illustrations of the mechanisms in photoexcited charge transfer from BFO films to the electrolyte and from excited surface modifiers to the BFO films after the BFO films were (c) positively and (d) negatively poled. Reprinted from Ref. [
Since there are many inherent limitations in the light absorption and the catalytic performance of the ferroelectric perovskite materials when they are used alone for solar water splitting, many recent studies that using ferroelectric material as a part of the heterojunction structures have been reported. According to the previous studies, the tunable remnant ferroelectric polarization in the ferroelectric material of the ferroelectric-based heterojunction could redistribute the charge carriers in the ferroelectric materials and its adjacent semiconductor. Thus, utilizing tunable ferroelectric polarization of ferroelectric material could change the band structure and modulate the performance of the ferroelectric-based heterojunction[
4.4. Construction of heterojunction structure
The approach of construction of heterojunction structure is another effective strategy for improving photocatalytic activity of perovskite materials for solar water splitting. A suitable heterojunction nano-architecture, where the formed band structure is favorable for the charge carriers generation in one semiconductor and transport them to the adjacent materials vectorially, which can result in efficient charge separation and fast charge migration, and can expand the light absorption range of the wide bandgap perovskite materials when combining them with a narrow bandgap semiconductor[
Figure 10.(Color online) Schematic illustration of the (a) electron–hole separation of La2Ti2O7/LaCrO3 heterojunction and (b) g-C3N4/SrTiO3:Rh composite under visible light irradiation. Reprinted from Refs. [
Some new strategies that systematically investigate the synergistic effects of heterojunction and other modifications such as changing of crystal structure and doping of foreign elements have been used to construct perovskite based catalysts for water splitting reaction. For example, Jia et al. constructed a BiVO4-Ru/SrTiO3:Rh composite Z-scheme photocatalyst by an impregnation method and a liquid-solid state reaction. The suitable contact was formed between BiVO4 and Ru/SrTiO3:Rh, which resulted in efficient electron transfer at interface, and the present composite photocatalyst demonstrated a high activity for water splitting under visible light irradiation. The indepth study showed that the crystallinity and morphology of BiVO4 could also have effects on the photocatalytic activity of the composite[
In addition to the construction of Z-scheme photocatalyst, using the effect of structure engineering and heterojunction synergistically can also improve the activity of photocatalyst. As shown in Fig. 11, Wei et al. constructed a hollow multi-shelled (HoMs) SrTiO3/TiO2 heterojunction by a hydrothermal crystallization method. The broccoli-like SrTiO3/TiO2 heterogeneous HoMs structures exhibited a fourfold higher overall water splitting performance than that of bare SrTiO3, which could be attributed to the special HoMs structures and the fabricated heterojunctions. It was reported that the HoMs structures could increase the light absorption ability of the composite photocatalysts and the constructed heterojunctions could boost the transfer and separation efficiency of the photogenerated electrons and holes. Therefore, the HoMs structures are helpful for enhancing the photocatalytic activity of the SrTiO3/TiO2 heterojunctions[
Figure 11.(Color online) (a) Synthesis route of SrTiO3/TiO2 HoMs from hydrothermal reaction. (b) Typical time course of photocatalytic H2 and O2 evolution reaction of triple shelled SrTiO3/TiO2 HoMs photocatalyst. (c) Possible band structure diagram for SrTiO3/TiO2 HoMs. Reprinted from Ref. [
4.5. Construction of plasmonic-based photocatalysts
The decoration of semiconductors with plasmonic metals and construction of plasmonic-based photocatalysts is another promising way to improve the photocatalytic performance of photocatalysts for solar water splitting. Generally, there are four main mechanisms of plasmonic effects on the photocatalytic process of solar water splitting, including: i) the improvement of light absorption efficiency by either trapping or scattering light into the semiconductors using the plasmonic metal nanoparticles (NPs); ii) hot-electrons injection; iii) plasmon-induced resonance energy transfer (PIRET) and iv) the role of cocatalysis[
Figure 12.(Color online) Schematic illustration of (a) the photogenerated charge transfer and catalytic reaction mechanisms of the fabricated LaCoO3/Au nano-composite (Reprinted from Ref. [
Additionally, some studies on using the synergistic effect of plasmonic enhancement and other strategies have been reported to improve the activity of catalyst to an unprecedentedly high level for solar water splitting. Our group has combined the plasmon resonance effect with the ferroelectric enhancement, and designed a plasmonic-ferroeletric hybrid PEC photoelectrode. As shown in Fig. 13, we explored the charge transfer and transport in nano-Au/PZT hybrids by constructing three nanostructures including ITO/Au/PZT, ITO/PZT/Au/PZT, and ITO/PZT/Au, and by poling the PZT films with different potentials. Due to the LSPR effect of the nano-Au, the nano-Au/PZT hybrids could broaden the light absorbance to the NIR range (800 nm). The spatial distribution of the electric field intensity around the square dot was obtained by the finite difference time domain (FDTD) method under 800 nm light illumination. It indicated that the strong field was located at the edge of the Au square and the hot spots might increase the probability of the hot charge carriers transfer from Au to the nearby materials. Moreover, using the ITO/PZT/nano-Au/PZT electrodes, we demonstrated the tunable photocurrent by changing the pre-poling bias from –10 to +10 V. This is attributed to the ferroeletric performance of the PZT, which can form different band bending at PZT/electrolyte interface under different pre-poling bias and affect the hot electron transfer from Au to PZT. The femtosecond transient absorbance results even more confirmed the hot charge transfer from Au NPs to PZT[
Figure 13.(Color online) (a) Schematics and cross-section of scanning electron microscope (SEM) of the fabricated ITO/PZT/Au, ITO/PZT/Au/PZT, and ITO/Au/PZT electrodes. (b) Top-view SEM image of nano-Au array fabricated on ITO glass; scale bar, 1
5. Conclusions and perspectives
In this review, we reviewed recent progress and the design strategies in the field of solar water splitting based on the perovskite materials. Our aims were to provide some useful guidance for future research on the design of perovskite-based photocatalysts and systems for water splitting reaction. Although some great progress in the construction of perovskite-based photocatalysts and systems for water splitting have also been made by implementing different design strategies, such as adjustment of the chemical component, crystal structure and morphology engineering, application of ferroelectric property, construction of heterojunction structure and plasmonic-based photocatalysts, etc. However, the STH conversion efficiency of the current water splitting systems is still very low (about 2%) for large-scale applications of H2 generation[
(1) The selection and doping of A, B and X sites elements in the perovskite are proved to effectively reduce the band gap and improve the catalytic activity. However, the low light harvesting ability is still a critical problem of most perovskite-based catalysts for solar water splitting. The development of experimental techniques are needed to precisely control the position, element and concentration of the dopants. Moreover, co-doping of metal and non-metal elements would be a useful strategy to modify the band structures of perovskites to meet the highly efficient solar water splitting.
(2) Surface engineering, crystal structure, morphology engineering, and construction of heterojunction structure have been proved to be four valid strategies for modifying perovskite materials to improve the photo/photoelectrocatalytic activity by facilitating surface reactions, suppressing charge carriers recombination, reducing the particle size and increasing the surface areas, etc. However, the interaction mechanism and the synergetic effect of the different components of the perovskite-based composites have not been clearly addressed. The further efforts such as using the new experimental methods and theoretical calculations should be taken to clarify the problem, which could be the effective directions.
(3) There is an activation barrier for H2 and O2 evolution reactions and most of the perovskite based photocatalysts are broad-band gap materials (> 2 eV), thus, designing and finding the optimal photocatalyst with the best activity and suitable band structure is still the challenge for overall water splitting. Therefore, much more efforts should be taken to develop the photocatalyst with multifunctionality that has wide light absorption range, efficient photogenerated charge carriers separation ability, sufficient catalytic active sites, high stability, and appropriate band structure for water redox reactions. Moreover, utilization of different enhancement effects and some advanced synthetic techniques synergistically to develop the high efficiency photocatalysts and systems could be an effective direction.
(4) In addition to the development of photocatalysts and systems with high activity, systematic investigation of the working mechanisms and reaction mechanisms of the perovskite based photo/photoelectrocatalytic systems should be another critical issue to be addressed. The comprehensively experimental and theoretical studies should be designed and conducted to investigate the intrinsic mechanism of the system in the future, especially for the compound photocatalysts and the complex systems. It may be beneficial for the future research and can provide useful guidance for design of perovskite based photocatalytic system with superior photo/photoelectrocatalytic activity and stability. Therefore, more efficient experimental and computational methods need to be developed in the future to overcome these problems.
Acknowledgement
This work was mostly supported by National Natural Science Foundation of China (Grant No. 21975245, 51972300 and 61674141), Key Research Program of Frontier Science, CAS (Grant No. QYZDB-SSW-SLH006), the National Key Research and Development Program of China (Grant No. 2017YFA0206600, 2018YFE0204000), the National Basic Research Program of China (Grant No. 2014CB643503), Z. W. appreciates the support from Hundred-Talent Program (Chinese Academy of Sciences).
References
[1] S E Hosseini, M A Wahid. Hydrogen production from renewable and sustainable energy resources: Promising green energy carrier for clean development. Renew Sust Energ Rev, 57, 850(2016).
[2] A Vita, C Italiano, L Pino et al. Hydrogen-rich gas production by steam reforming of n-dodecane. Part II: Stability, regenerability and sulfur poisoning of low loading Rh-based catalyst. Appl Catal B, 218, 317(2017).
[3] T Hisatomi, K Domen. Reaction systems for solar hydrogen production via water splitting with particulate semiconductor photocatalysts. Nat Catal, 2, 387(2019).
[4] W Wang, M Xu, X Xu et al. Perovskite oxide-based electrodes for high-performance photoelectrochemical water splitting. Angew Chem Int Ed Engl, 58, 2(2019).
[5] G Chen, Z Hu, Y Zhu et al. A universal strategy to design superior water-splitting electrocatalysts based on fast in situ reconstruction of amorphous nanofilm precursors. Adv Mater, 30, 1804333(2018).
[6] G Zhang, G Liu, L Z Wang et al. Inorganic perovskite photocatalysts for solar energy utilization. Chem Soc Rev, 45, 5951(2016).
[7] X Sheng, T Xu, X J Feng. Rational design of photoelectrodes with rapid charge transport for photoelectrochemical applications. Adv Mater, 31, 1805132(2019).
[8] W J Chen, T T Wang, J W Xue et al. Cobalt-nickel layered double hydroxides modified on TiO2 nanotube arrays for highly efficient and stable PEC water splitting. Small, 13, 1602420(2017).
[9] M Faraji, M Yousefi, S Yousefzadeh et al. Two-dimensional materials in semiconductor photoelectrocatalytic systems for water splitting. Energ Environ Sci, 12, 59(2019).
[10] X Huang, X Y Qi, F Boey et al. Graphene-based composites. Chem Soc Rev, 41, 666(2012).
[11] X Li, S W Liu, K Fan et al. MOF-based transparent passivation layer modified ZnO nanorod arrays for enhanced photo-electrochemical water splitting. Adv Energy Mater, 8, 1800101(2018).
[12] W J Ong, L L Tan, Y H Ng et al. Graphitic carbon nitride (g-C3N4)-based photocatalysts for artificial photosynthesis and environmental remediation: are we a step closer to achieving sustainability. Chem Rev, 116, 7159(2016).
[13] S C Wang, P Chen, Y Bai et al. New BiVO4 dual photoanodes with enriched oxygen vacancies for efficient solar-driven water splitting. Adv Mater, 30, 1800486(2018).
[14] Y F Xu, H S Rao, B X Chen et al. Achieving highly efficient photoelectrochemical water oxidation with a TiCl4 treated 3D antimony-doped SnO2 macropore/branched alpha-Fe2O3 nanorod heterojunction photoanode. Adv Sci, 2, 1500049(2015).
[15] T F Yeh, C Y Teng, L C Chen et al. Graphene oxide-based nanomaterials for efficient photoenergy conversion. J Mater Chem A, 4, 2014(2016).
[16] Z M Zhang, C T Gao, Z M W u et al. Toward efficient photoelectrochemical water-splitting by using screw-like SnO2 nanostructures as photoanode after being decorated with CdS quantum dots. Nano Energy, 19, 318(2016).
[17] Y E Zhou, L Y Zhang, L H Lin et al. Highly efficient photoelectrochemical water splitting from hierarchical WO3/BiVO4 nanoporous sphere arrays. Nano Lett, 17, 8012(2017).
[18] B A Pinaud, J D Benck, L C Seitz et al. Technical and economic feasibility of centralized facilities for solar hydrogen production via photocatalysis and photoelectrochemistry. Energ Environ Sci, 6, 1983(2013).
[19] P Tan, M L Liu, Z P Shao et al. Recent advances in perovskite oxides as electrode materials for nonaqueous lithium-oxygen batteries. Adv Energy Mater, 7, 1602674(2017).
[20] W Wang, M O Tade, Z P Shao. Nitrogen-doped simple and complex oxides for photocatalysis: A review. Prog Mater Sci, 92, 33(2018).
[21] M A Pena, J L Fierro. Chemical structures and performance of perovskite oxides. Chem Rev, 101, 1981(2001).
[22] J Suntivich, K J May, H A Gasteiger et al. A perovskite oxide optimized for oxygen evolution catalysis from molecular orbital principles. Science, 334, 1383(2011).
[23] J Hwang, R R Rao, L Giordano et al. Perovskites in catalysis and electrocatalysis. Science, 358, 751(2017).
[24] Z Y Cheng, J Lin. Layered organic-inorganic hybrid perovskites: structure, optical properties, film preparation, patterning and templating engineering. CrystEngComm, 12, 2646(2010).
[25] M A Green, A Ho-Baillie, H J Snaith. The emergence of perovskite solar cells. Nat Photonics, 8, 506(2014).
[26] V M Goldschmidt. Crystal structure and chemical correlation. Ber Dtsch Chem Ges, 60, 1263(1927).
[27] W Wang, M O Tade, Z P Shao. Research progress of perovskite materials in photocatalysis- and photovoltaics-related energy conversion and environmental treatment. Chem Soc Rev, 44, 5371(2015).
[28] C Li, K C K Soh, P Wu. Formability of ABO3 perovskites. J Alloy Compd, 372, 40(2004).
[29] C H Li, X G Lu, W Z Ding et al. Formability of ABX3 (X = F, Cl, Br, I) halide perovskites. Acta Crystallogr B, 64, 702(2008).
[30] C C Hu, Y L Lee, H S Teng. Efficient water splitting over Na1–
[31] P Li, S X Ouyang, G C Xi et al. The effects of crystal structure and electronic structure on photocatalytic H2 evolution and CO2 reduction over two phases of perovskite-structured NaNbO3. J Phys Chem C, 116, 7621(2012).
[32] R E Cohen. Origin of ferroelectricity in perovskite oxides. Nature, 358, 136(1992).
[33] A Ohtomo, H Y Hwang. A high-mobility electron gas at the LaAlO3/SrTiO3 heterointerface. Nature, 427, 423(2004).
[34] N Reyren, S Thiel, A D Caviglia et al. Superconducting interfaces between insulating oxides. Science, 317, 1196(2007).
[35] S Jin, T H Tiefel, M McCormack et al. Thousandfold change in resistivity in magnetoresistive La–Ca–Mn–O films. Science, 264, 413(1994).
[36] K Huang, R S Tichy, J B Goodenough. Superior perovskite oxide-Ion conductor; strontium- and magnesium-doped LaGaO3:I, phase relationships and electrical properties. J Am Ceram Soc, 81, 2565(1998).
[37] J Ibarra. Influence of composition on the structure and conductivity of the fast ionic conductors La2/3−
[38] K S Chan, J Ma, S Jaenicke et al. Catalytic carbon-monoxide oxidation over strontium, cerium and copper-substituted lanthanum manganates and cobaltates. Appl Catal A, 107, 201(1994).
[39] S Royer, D Duprez, F Can et al. Perovskites as substitutes of noble metals for heterogeneous catalysis: dream or reality. Chem Rev, 114, 10292(2014).
[40] W J Yin, B Weng, J Ge et al. Oxide perovskites, double perovskites and derivatives for electrocatalysis, photocatalysis, and photovoltaics. Energ Environ Sci, 12, 442(2019).
[41] D Mignard, R C Batik, A S Bharadwaj et al. Revisiting strontium-doped lanthanum cuprate perovskite for the electrochemical reduction of CO2. J CO2 Util, 5, 53(2014).
[42] J Suntivich, H A Gasteiger, N Yabuuchi et al. Design principles for oxygen-reduction activity on perovskite oxide catalysts for fuel cells and metal-air batteries. Nat Chem, 3, 546(2011).
[43] A Fujishima, K Honda. Electrochemical photolysis of water at a semiconductor electrode. Nature, 238, 37(1972).
[44] A Kudo, Y Miseki. Heterogeneous photocatalyst materials for water splitting. Chem Soc Rev, 38, 253(2009).
[45] S S Chen, T Takata, K Domen. Particulate photocatalysts for overall water splitting. Nat Rev Mater, 2, 17050(2017).
[46] J H Kim, D Hansora, P Sharma et al. Toward practical solar hydrogen production-an artificial photosynthetic leaf-to-farm challenge. Chem Soc Rev, 48, 1908(2019).
[47] M Q Yang, M M Gao, M H Hong et al. Visible-to-NIR photon harvesting: progressive engineering of catalysts for solar-powered environmental purification and fuel production. Adv Mater, 30, 1802894(2018).
[48] X B Li, C H Tung, L Z Wu. Semiconducting quantum dots for artificial photosynthesis. Nat Rev Chem, 2, 160(2018).
[49] K Maeda, K Domen. New non-oxide photocatalysts designed for overall water splitting under visible light. J Phys Chem C, 111, 7851(2007).
[50] R M N Yerga, M C A Galvan, F del Valle et al. Water splitting on semiconductor catalysts under visible-light irradiation. ChemSusChem, 2, 471(2009).
[51] A P Pushkarev, M N Bochkarev. Organic electroluminescent materials and devices emitting in UV and NIR regions. Russ Chem Rev, 85, 1338(2016).
[52] Z Kang, H N Si, S C Zhang et al. Interface engineering for modulation of charge carrier behavior in ZnO photoelectrochemical water splitting. Adv Funct Mater, 29, 1808032(2019).
[53] C R Jiang, S J A Moniz, A Q Wang et al. Photoelectrochemical devices for solar water splitting-materials and challenges. Chem Soc Rev, 46, 4645(2017).
[54] M Kitano, M Takeuchi, M Matsuoka et al. Photocatalytic water splitting using Pt-loaded visible light-responsive TiO2 thin film photocatalysts. Catal Today, 120, 133(2007).
[55] N T Suen, S F Hung, Q Quan et al. Electrocatalysis for the oxygen evolution reaction: recent development and future perspectives. Chem Soc Rev, 46, 337(2017).
[56] P Kanhere, Z Chen. A review on visible light active perovskite-based photocatalysts. Molecules, 19, 19995(2014).
[57] M Moniruddin, B Ilyassov, X Zhao et al. Recent progress on perovskite materials in photovoltaic and water splitting applications. Mater Today Energy, 7, 246(2018).
[58] M A Khan, M A Nadeem, H Idrissn. Ferroelectric polarization effect on surface chemistry and photo-catalytic activity: A review. Surf Sci Rep, 71, 1(2016).
[59] R Konta, T Ishii, H Kato et al. Photocatalytic activities of noble metal ion doped SrTiO3 under visible light irradiation. J Phys Chem B, 108, 8992(2004).
[60] T Ohno, T Tsubota, Y Nakamura et al. Preparation of S, C cation-codoped SrTiO3 and its photocatalytic activity under visible light. Appl Catal A, 288, 74(2005).
[61] E Grabowska. Selected perovskite oxides: characterization, preparation and photocatalytic properties-A review. Appl Catal B, 186, 97(2016).
[62] M Kawasaki, K Takahashi, T Maeda et al. Atomic control of the SrTiO3 crystal surface. Science, 266, 1540(1994).
[63] K Iwashina, A Kudo. Rh-doped SrTiO3 photocatalyst electrode showing cathodic photocurrent for water splitting under visible-light irradiation. J Am Chem Soc, 133, 13272(2011).
[64] U S Shenoy, H Bantawal, D K Bhat. Band engineering of SrTiO3: effect of synthetic technique and site occupancy of doped rhodium. J Phys Chem C, 122, 27567(2018).
[65] T Umebayashi, T Yamaki, H Itoh et al. Analysis of electronic structures of 3d transition metal-doped TiO2 based on band calculations. J Phys Chem Solids, 63, 1909(2002).
[66] J P Zou, L Z Zhang, S L Luo et al. Preparation and photocatalytic activities of two new Zn-doped SrTiO3 and BaTiO3 photocatalysts for hydrogen production from water without cocatalysts loading. Int J Hydrogen Energ, 37, 17068(2012).
[67] M Machida, K Miyazaki, S Matsushima et al. Photocatalytic properties of layered perovskite tantalates, MLnTa2O7 (M = Cs, Rb, Na, and H; Ln = La, Pr, Nd, and Sm). J Mater Chem, 13, 1433(2003).
[68] J Yin, Z Zou, J Ye. Photophysical and photocatalytic properties of MIn0.5Nb0.5O3 (M = Ca, Sr, and Ba). J Phys Chem B, 107, 61(2003).
[69] B B Dong, J Y Cui, T F Liu et al. Development of novel perovskite-like oxide photocatalyst LiCuTa3O9 with dual functions of water reduction and oxidation under visible light irradiation. Adv Energy Mater, 8, 1801660(2018).
[70] B Wang, P D Kanhere, Z Chen et al. Anion-doped NaTaO3 for visible light photocatalysis. J Phys Chem C, 117, 22518(2013).
[71] F F Li, D R Liu, G M Gao et al. Improved visible-light photocatalytic activity of NaTaO3 with perovskite-like structure via sulfur anion doping. Appl Catal B, 166/167, 104(2015).
[72] H Yu, J J Wang, S C Yan et al. Elements doping to expand the light response of SrTiO3. J Photoch Photobio A, 275, 65(2014).
[73] M Humayun, L Xu, L Zhou et al. Exceptional co-catalyst free photocatalytic activities of B and Fe co-doped SrTiO3 for CO2 conversion and H2 evolution. Nano Res, 11, 6391(2018).
[74] C Pan, T Takata, K Kumamoto et al. Band engineering of perovskite-type transition metal oxynitrides for photocatalytic overall water splitting. J Mater Chem A, 4, 4544(2016).
[75] J Shi, J Ye, Z Zhou et al. Hydrothermal synthesis of Na0.5La0.5TiO3-LaCrO3 solid-solution single-crystal nanocubes for visible-light-driven photocatalytic H2 evolution. Chem-Eur J, 17, 7858(2011).
[76] D Wang, T Kako, J Ye. Efficient photocatalytic decomposition of acetaldehyde over a solid-solution perovskite (Ag0.75Sr0.25)(Nb0.75Ti0.25)O3 under visible-light irradiation. J Am Chem Soc, 130, 2724(2008).
[77] W Luo, Z Li, X Jiang et al. Correlation between the band positions of (SrTiO3)1−
[78] S Cho, J W Jang, W Zhang et al. Single-crystalline thin films for studying intrinsic properties of BiFeO3-SrTiO3 solid solution photoelectrodes in solar energy conversion. Chem Mater, 27, 6635(2015).
[79] L Lu, M Lv, D Wang et al. Efficient photocatalytic hydrogen production over solid solutions Sr1–
[80] G Zhang, S Sun, W Jiang et al. A novel perovskite SrTiO3-Ba2FeNbO6 solid solution for visible light photocatalytic hydrogen production. Adv Energy Mater, 7, 1600932(2017).
[81] W Li, K Jiang, Z Li et al. Origin of improved photoelectrochemical water splitting in mixed perovskite oxides. Adv Mater, 8, 1801972(2018).
[82] D J Martin, N Umezawa, X Chen et al. Facet engineered Ag3PO4 for efficient water photooxidation. Energ Environ Sci, 6, 3380(2013).
[83] D J Martin, K Qiu, S A Shevlin et al. Highly efficient photocatalytic H2 evolution from water using visible light and structure-controlled graphitic carbon nitride. Angew Chem Int Ed, 53, 9240(2014).
[84] Y Ham, T Hisatomi, Y Goto et al. Flux-mediated doping of SrTiO3 photocatalysts for efficient overall water splitting. J Mater Chem A, 4, 3027(2016).
[85] L Mu, Y Zhao, A Li et al. Enhancing charge separation on high symmetry SrTiO3 exposed with anisotropic facets for photocatalytic water splitting. Energ Environ Sci, 9, 2463(2016).
[86] D L Zhong, W W Liu, P F Tan et al. Insights into the synergy effect of anisotropic {001} and {230} facets of BaTiO3 nanocubes sensitized with CdSe quantum dots for photocatalytic water reduction. Appl Catal B, 227, 1(2018).
[87] M Qiao, J Liu, Y Wang et al. PdSeO3 monolayer: promising inorganic 2D photocatalyst for direct overall water splitting without using sacrificial reagents and cocatalysts. J Am Chem Soc, 140, 12256(2018).
[88] S Chandrasekaran, E J Kim, J S Chung et al. Structurally tuned lead magnesium titanate perovskite as a photoelectrode material for enhanced photoelectrochemical water splitting. Chem Eng J, 309, 682(2017).
[89] K M Parida, K H Reddy, S Martha et al. Fabrication of nanocrystalline LaFeO3: An efficient sol-gel auto-combustion assisted visible light responsive photocatalyst for water decomposition. Int J Hydrogen Energ, 35, 12161(2010).
[90] S N Tijare, M V Joshi, P S Padole et al. Photocatalytic hydrogen generation through water splitting on nano-crystalline LaFeO3 perovskite. Int J Hydrogen Energ, 37, 10451(2012).
[91] C W Lee, D W Kim, I S Cho et al. Simple synthesis and characterization of SrSnO3 nanoparticles with enhanced photocatalytic activity. Int J Hydrogen Energ, 37, 10557(2012).
[92] M Klusackova, R Nebel, K M Macounova et al. Size control of the photo-electrochemical water splitting activity of SrTiO3 nano-cubes. Electrochimica Acta, 297, 215(2019).
[93] A Kudo, A Tanaka, K Domen et al. The effects of the calcination temperature of SrTiO3 powder on photocatalytic activities. J Catal, 111, 296(1988).
[94] I Grinberg, D V West, M Torres et al. Perovskite oxides for visible-light-absorbing ferroelectric and photovoltaic materials. Nature, 503, 509(2013).
[95] H T Yi, T Choi, S G Choi et al. Mechanism of the switchable photovoltaic effect in ferroelectric BiFeO3. Adv Mater, 23, 3403(2011).
[96] A Bhatnagar, A R Chaudhuri, Y H Kim et al. Role of domain walls in the abnormal photovoltaic effect in BiFeO3. Nat Commun, 4, 2835(2013).
[97] D Cao, J Xu, L Fang et al. Interface effect on the photocurrent: A comparative study on Pt sandwiched (Bi3.7Nd0.3)Ti3O12 and Pb(Zr0.2Ti0.8)O3 films. Appl Phys Lett, 96, 192101(2010).
[98] C Wang, D Cao, F Zheng et al. Photocathodic behavior of ferroelectric Pb(Zr,Ti)O3 films decorated with silver nanoparticles. Chem Commun, 49, 3769(2013).
[99] D Cao, Z Wang et al. Switchable charge-transfer in the photoelectrochemical energy-conversion process of ferroelectric BiFeO3 photoelectrodes. Angew Chem Int Ed, 53, 11027(2014).
[100] J Song, T L Kim, J Lee et al. Domain-engineered BiFeO3 thin-film photoanodes for highly enhanced ferroelectric solar water splitting. Nano Res, 11, 642(2018).
[101] Z Wang, D Cao, L Wen et al. Manipulation of charge transfer and transport in plasmonic-ferroelectric hybrids for photoelectrochemical applications. Nat Commun, 7, 10348(2016).
[102] J Shi, P Zhao, X Wang. Piezoelectric-polarization-enhanced photovoltaic performance in depleted-heterojunction quantum-dot solar cells. Adv Mater, 25, 916(2013).
[103] X Huang, K Wang, Y Wang et al. Enhanced charge carrier separation to improve hydrogen production efficiency by ferroelectric spontaneous polarization electric field. Appl Catal B, 227, 322(2018).
[104] W Yang, Y Yu, M B Starr et al. Ferroelectric polarization-enhanced photoelectrochemical water splitting in TiO2-BaTiO3 core-shell nanowire photoanodes. Nano Lett, 15, 7574(2015).
[105] W Li, F Wang, M Li et al. Polarization-dependent epitaxial growth and photocatalytic performance of ferroelectric oxide heterostructures. Nano Energy, 45, 304(2018).
[106] A A Iyer, E Ertekin. Asymmetric response of ferroelectric/metal oxide heterojunctions for catalysis arising from interfacial chemistry. Phys Chem Chem Phys, 19, 5870(2017).
[107] J H Lee, A Selloni. TiO2/ferroelectric heterostructures as dynamic polarization-promoted catalysts for photochemical and electrochemical oxidation of water. Phys Rev Lett, 112, 196102(2014).
[108] J Xie, C Guo, P Yang et al. Bi-functional ferroelectric BiFeO3 passivated BiVO4 photoanode for efficient and stable solar water oxidation. Nano Energy, 31, 28(2017).
[109] J Low, J Yu, M Jaroniec et al. Heterojunction photocatalysts. Adv Mater, 29, 1601694(2017).
[110] H Li, Y Zhou, W Tu et al. State-of-the-art progress in diverse heterostructured photocatalysts toward promoting photocatalytic performance. Adv Funct Mater, 25, 998(2015).
[111] A Nashim, K Parida. n-La2Ti2O7/p-LaCrO3: a novel heterojunction based composite photocatalyst with enhanced photoactivity towards hydrogen production. J Mater Chem A, 2, 18405(2014).
[112] X Xu, G Liu, C Randorn et al. g-C3N4 coated SrTiO3 as an efficient photocatalyst for H2 production in aqueous solution under visible light irradiation. Int J Hydrogen Energ, 36, 13501(2011).
[113] H W Kang, S N Lim, D Song et al. Organic-inorganic composite of g-C3N4-SrTiO3:Rh photocatalyst for improved H2 evolution under visible light irradiation. Int J Hydrogen Energ, 37, 11602(2012).
[114] F Opoku, K K Govender, C G C E van Sittert et al. Tuning the electronic structures, work functions, optical properties and stability of bifunctional hybrid graphene oxide/V-doped NaNbO3 type-II heterostructures: A promising photocatalyst for H2 production. Carbon, 136, 187(2018).
[115] Q Jia, A Iwase, A Kudo. BiVO4-Ru/SrTiO3:Rh composite Z-scheme photocatalyst for solar water splitting. Chem Sci, 5, 1513(2014).
[116] C Dong, S Lu, S Yao et al. Colloidal synthesis of ultrathin monoclinic BiVO4 nanosheets for Z-scheme overall water splitting under visible light. ACS Catal, 8, 8649(2018).
[117] Z Ma, Y Li, Y Lv et al. Synergistic effect of doping and compositing on photocatalytic efficiency: a case study of La2Ti2O7. ACS Appl Mater Inter, 10, 39327(2018).
[118] Y Wei, J Wang, R Yu et al. Constructing SrTiO3-TiO2 heterogeneous hollow multi-shelled structures for enhanced solar water splitting. Angew Chem Int Ed, 131, 1436(2019).
[119] Y Chang, K Yu, C Zhang et al. Ternary CdS/Au/3DOM-SrTiO3 composites with synergistic enhancement for hydrogen production from visible-light photocatalytic water splitting. Appl Catal B, 215, 74(2017).
[120] M Valenti, M P Jonsson, G Biskos et al. Plasmonic nanoparticle-semiconductor composites for efficient solar water splitting. J Mater Chem A, 4, 17891(2016).
[121] P Zhang, T Wang, J Gong. Mechanistic understanding of the plasmonic enhancement for solar water splitting. Adv Mater, 27, 5328(2015).
[122] D Xu, S Yang, Y Jin et al. Ag-decorated ATaO3 (A = K, Na) nanocube plasmonic photocatalysts with enhanced photocatalytic water-splitting properties. Langmuir, 31, 9694(2015).
[123] J Liu, Y Sun, Z Li et al. Photocatalytic hydrogen production from water/methanol solutions over highly ordered Ag-SrTiO3 nanotube arrays. Int J Hydrogen Energ, 36, 5811(2011).
[124] D Lu, S Ouyang, H Xu et al. Designing Au surface-modified nanoporous-single-crystalline SrTiO3 to optimize diffusion of surface plasmon resonance-induce photoelectron toward enhanced visible-light photoactivity. ACS Appl Mater Inter, 8, 9506(2016).
[125] B T Zhang, J Liu, S Yue et al. Hot electron injection: an efficacious approach to charge LaCoO3 for improving the water splitting efficiency. Appl Catal B, 219, 432(2017).
[126] Y B Huang, J Liu, D W Cao et al. Separation of hot electrons and holes in Au/LaFeO3 to boost the photocatalytic activities both for water reduction and oxidation. Int J Hydrogen Energ, 44, 13242(2019).
[127] X Cai, M Zhu, O A Elbanna et al. Au nanorod photosensitized La2Ti2O7 nanosteps: successive surface heterojunctions boosting visible to near-infrared photocatalytic H2 evolution. ACS Catal, 8, 122(2018).
[128] L Shi, W Zhou, Z Li et al. Periodically ordered nanoporous perovskite photoelectrode for efficient photoelectrochemical water splitting. ACS Nano, 12, 6335(2018).
[129] Y Zhong, K Ueno, Y Mori et al. Plasmon-assisted water splitting using two sides of the same SrTiO3 single-crystal substrate: conversion of visible light to chemical energy. Angew Chem Int Ed, 53, 10350(2014).
[130] Q Liu, Y Zhou, L You et al. Enhanced ferroelectric photoelectrochemical properties of polycrystalline BiFeO3 film by decorating with Ag nanoparticles. Appl Phys Lett, 108, 022902(2016).
[131] Y L Huang, W S Chang, C N Van et al. Tunable photoelectrochemical performance of Au/BiFeO3 heterostructure. Nanoscale, 8, 15795(2016).
[132] M Zhu, X Cai, M Fujitsuka et al. Au/La2Ti2O7 nanostructures sensitized with black phosphorus for plasmon-enhanced photocatalytic hydrogen production in visible and near-infrared light. Angew Chem Int Ed, 56, 2064(2017).
[133] J Liu, Y Liu, N Liu et al. Metal-free efficient photocatalyst for stable visible water splitting via a two-electron pathway. Science, 347, 970(2015).
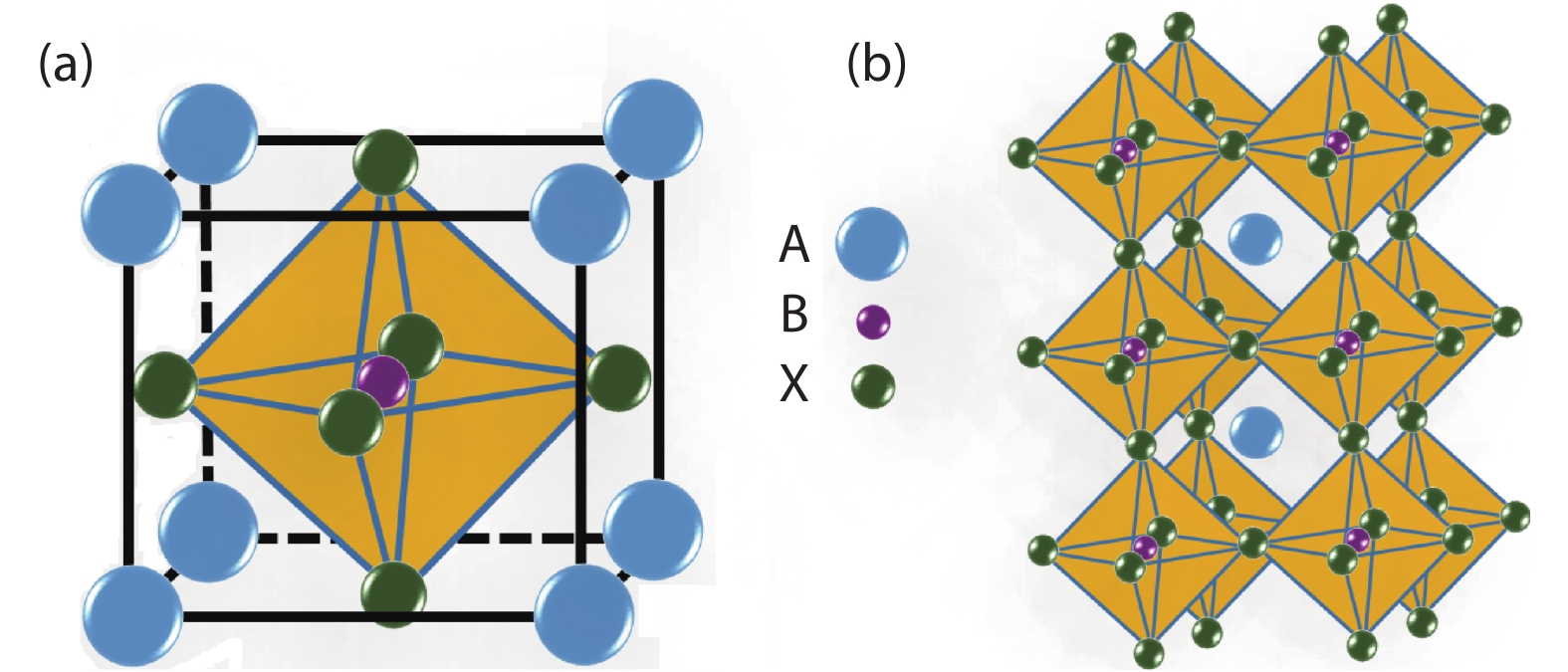
Set citation alerts for the article
Please enter your email address