
- Opto-Electronic Advances
- Vol. 3, Issue 12, 200023-1 (2020)
Abstract
Introduction
Generation and control of an ultrafast spin-photocurrent in topological materials are of great significance
Nodal-line semimetals (NLSs), which host one-dimensional closed-loop or line degeneracies formed by the crossing of two bands, have been proposed recently as a family of topological phase materials
In this paper, we exploit the chiral-controlled spin photocurrent in nodal line semimetal candidate Mg3Bi2 through terahertz emission spectroscopy. By investigating the emitted THz electromagnetic wave under the photon injection, we found the polarity and chirality of the photocurrents can be manipulated by polarized near-infrared optical pump on a picosecond timescale. In addition, we demonstrate that the chirality-dependent terahertz emission is mainly originated from the circular photogalvanic effect (CPGE), which is associated with the angular momentum selection rules of topological surface states.
Results and discussion
Materials growth and characterization
Mg3Bi2 is a layered Kagome lattice structure with the space group of
Figure 1.Characterization of the Mg3Bi2 film.
Terahertz emission analysis
Terahertz time-domain spectroscopy (TDS) is a crucial method to confirm the properties of topological states and the dynamics of charge carriers on a sub-picosecond timescale
Here,
Figure 2.Schematic view of helicity-dependent terahertz radiation process.
In addition, THz spectroscopy is a viable contactless alternative that can complement conventional transport measurement without the necessity for electrodes or background signal that may disturb the system
The schematic of the experimental setup is shown in Fig. 3(a) and the diagram of our terahertz emission system is depicted in Fig. S1. The Mg3Bi2 thin film was set up in the transmission direction of the pump light. The optical pulses with a central wavelength of 800 nm (1.55 eV) were focused on the crystal surface at an incident angle of θ to generate ultrafast photocurrent and then the terahertz wave was radiated. The vertical and horizonal components (along the Ex and Ey direction) of the emitted THz pulses were detected by electro-optical sampling, respectively. The polarization and chirality of optical pulses are controlled by rotating a quarter-wave plate (QWP) with an angle of α. The impact from bulk transient currents of Mg3Bi2 may contribute to the terahertz emissions in out-of-plane (perpendicular to the surface of the samples) direction, and these can be canceled by polarization settings of terahertz detection
Figure 3.(
The helicity-dependent terahertz emissions along the vertical direction (Ex) were measured by rotating a quarter-wave plate with an angle of α at incident angle θ = -45° and +45°, respectively, which were shown in Fig. 3. The polar plots of terahertz waveforms (0~4.5 ps) in Figs. 3(b) and 3(c) illustrate a clear polarity reversal at different helicity of optical excitations. According to the polarization trajectory, the polarization and chirality of the THz wave can be manipulated by the switching of circular or linear polarization of the incident light. Such controllability stems from the chiral ultrafast photocurrents derived from the polarized femtosecond optical pulses. Twofold symmetry was observed in the α-scan patterns, which gives more profound insights into the origins of terahertz emissions.
Figures 3(d) and 3(e) show the time domain terahertz waveforms of the Mg3Bi2 thin film for incident angle θ = -45° and +45° respectively, with linearly polarized (LP, α = 0°, red line), right-hand circularly polarized (RHCP, α = 45°, black line), and left-hand circularly polarized (LHCP, α = 135°, blue line) optical excitations. The key observation is that signals obtained from the right and left-handed circularly polarized light are completely in opposite phase for both θ = -45° and +45°, and for helicity-fixed optical excitation (both RHCP and LHCP), a similar polarity reversal was also found when the polarized light incident angle θ alternates from -45° in Fig. 3(d) to 45° in Fig. 3(e). This phenomenon usually results from the reversed incident photon helicity. THz signals from the linearly polarized light were also in the opposite phase for θ = -45° and +45°. Obviously, both the magnitude and temporal shape of the THz waveform depend strongly on the light polarization and the incident angle. These results are consistent with the scenario for the helicity-dependent terahertz emission: the spin-polarized current generated by incident photon polarization is the major contributor to this process
To further comprehend the origin of THz emission in Mg3Bi2, it is essential to figure out the mechanisms behind the generation of time-resolved photocurrent. We extracted the α-dependent THz amplitudes at the specific moment (t = 2.46 ps), which shows the largest variation of light response and can be controlled by rotating the QWP by an angle of α, as shown in Figs. 4(a) and 4(b). Based on the description of helicity-dependent photocurrents in topological insulators, the terahertz electric field can be inferred as ETHz(α, t)∝∂J(α, t)/∂t
Figure 4.(
In this equation, the coefficient C represents the contribution from helicity-dependent circular photogalvanic effect. L1 describes the helicity-independent linear photogalvanic effect
Based on our results, the amplitude and phase of Ex are dominated by C (CPGE, 34.87%) and L1 (LPGE, 51.72%), which were revealed in Figs. 4(c), 4(d) and Fig. S2. The extracted CPGE component of the emitted THz electric field follows a sinusoidal dependence of 2α whose periodicity matches a change for the spin direction of the incident light. However, the LPGE part shows a 4α periodicity because it has no response to the helicity of the incident ray. Both of them are coincident with the rotational symmetry of the Dirac cone of TIs
Terahertz signals in the Ey direction appear in a polarization-independent manner, which are substantially different from Ex, as shown in Fig. S3. On the one hand, signals in Ey direction are relatively weak compared with that in Ex direction. On the other hand, according to the fitting result, Ey is dominated by a polarization-independent D. L2 plays a minor role, while C and L1 can be neglected. These results suggest that the helicity-controlled terahertz emission mainly results from the polarization-dependent photocurrent in Ex direction, rather than the polarization-independent photocurrent in Ey direction. Such distinct features in Ex and Ey directions may lead to an elliptically polarized transient THz field
As another controllable measurement, we explore the relationship between the THz emission response and the power of incident optical pulses centered at 800 nm, with the polarization state fixed to right-hand circular polarization. It is apparent that the increase of the laser power augments the amplitude of the emitted THz waves but does not change the shape of the waveform, as shown in Fig. 5(a). Through fast Fourier transformations (FFT) of the THz waveforms, Figure 5(b) displays the frequency domain that covers the frequency range up to 2 THz. On the other hand, the peak value for all of the spectra is centered around 0.55 THz, which is in very good agreement with the results for the time-domain analysis. Additionally, as illustrated in Fig. 5(c), a linear behavior is indicated in the dependence of the THz peak amplitude on the incident pump power up to 1800 μJ/cm2. Figure 5(d) displays the azimuthal-scan (φ-scan) results for the peak-to-peak amplitudes of the terahertz radiations with linearly polarized optical pulses at nearly normal incidence (θ~0°) from Mg3Bi2 thin films. The azimuthal scan strongly depends on the crystal structure of samples. Obviously, Mg3Bi2 owns a centrosymmetric lattice structure for bulk, but the inversion symmetry of the top and bottom surface is broken. We deduce that the anisotropy of the azimuthal-scan results is related to the broken inversion symmetry of the surface.
Figure 5.(
To shed more light on the emission mechanism, terahertz radiation arising from the linearly polarized light can be further investigated. A half-wave plate is used to clarify the relationship between ETHz with the linear polarization angle α. The incident angle θ is fixed at 45°. The relationship between pump polarization angle and terahertz amplitude near the peak values is shown in Fig. 6(a). We found that ETHz induced by the linearly polarized light can be well described by a sinusoidal function with a period of 180°. The amplitude of the wave excited by linearly polarized light is approximately three times smaller than that of the wave excited by circularly polarized light, compared with Figs. 4(a) and 4(b). When the polarization angle of the pump beam α is changed from 0° to 90°, the polarity of the emitted THz pulse is reversed, as shown in Fig. 6(b). No THz emission is detected at a polarization angle of α = 45°. This phenomenon can be described by a second-order nonlinear optical process
Figure 6.(
Summary and discussions
In conclusion, for the first time, we demonstrated that spin photocurrent induced helicity-dependent terahertz emission could be realized in nodal-line semimetal candidate Mg3Bi2. The polarization directions and magnitudes of helical THz wave can be easily controlled without any THz waveplate. The polarization control is mainly originated from polarity-dependent photocurrent, and then can be effectively manipulated by circular photogalvanic effect. Such an intrinsic spintronic emitter shows promising characteristics and is easily accessible. Finally, this work will open up intriguing opportunities for fundamental studies of novel THz emission, THz spintronics, and THz modulation devices by using topological materials
Acknowledgements
We thank Prof. J. B. Qi for helpful discussions and are grateful for financial support from the National Natural Science Foundation of China (Grant Nos.11804387, 11802339, 11805276, 11902358, 61805282, and 61801498); the Scientific Researches Foundation of National University of Defense Technology (Grant Nos. ZK18-03-22, ZK18-01-03 and ZK18-03-36).
Author contributions
M. Y. Tong and Y. Z. Hu contributed equally to this work. J. Tian and M. Y. Tong conceived the idea and designed the research; M. Y. Tong and X. N. Xie fabricated all the samples; Y. Z. Hu performed the optical measurement. T. Jiang, Z. Y. Wang, X. A. Cheng and X. G. Zhu analysed the data; M. Y. Tong and Y. Z. Hu co-wrote the manuscript. All authors discussed and commented on the manuscript.
Competing interests
The authors declare no competing financial interests.
Supplementary information
Supplementary information for this paper is available at https://doi.org/10.29026/oea.2020.200023
References
[1] P Lodahl, S Mahmoodian, S Stobbe, A Rauschenbeutel, P Schneeweiss et al. Chiral quantum optics. Nature, 541, 473-480(2017).
[2] T Kampfrath, A Sell, G Klatt, A Pashkin, S Mährlein et al. Coherent terahertz control of antiferromagnetic spin waves. Nat Photonics, 5, 31-34(2011).
[3] S A Wolf, D D Awschalom, R A Buhrman, J M Daughton, S von Molnár et al. Spintronics: a spin-based electronics vision for the future. Science, 294, 1488-1495(2001).
[4] B Ferguson, X C Zhang. Materials for terahertz science and technology. Nat Mater, 1, 26-33(2002).
[5] J W McIver, D Hsieh, H Steinberg, P Jarillo-Herrero, N Gedik. Control over topological insulator photocurrents with light polarization. Nat Nanotechnol, 7, 96-100(2012).
[6] L Braun, G Mussler, A Hruban, M Konczykowski, T Schumann et al. Ultrafast photocurrents at the surface of the three-dimensional topological insulator Bi2Se3. Nat Commun, 7, 13259(2016).
[7] Y Gao, S Kaushik, E J Philip, Z Li, Y Qin et al. Chiral terahertz wave emission from the Weyl semimetal TaAs. Nat Commun, 11, 720(2020).
[8] Z L Zhang, Y P Chen, S Cui, F He, M Chen et al. Manipulation of polarizations for broadband terahertz waves emitted from laser plasma filaments. Nat Photonics, 12, 554-559(2018).
[9] A A Burkov, M D Hook, L Balents. Topological nodal semimetals. Phys Rev B, 84, 235126(2011).
[10] C Fang, Y G Chen, H Y Kee, L Fu. Topological nodal line semimetals with and without spin-orbital coupling. Phys Rev B, 92, 081201(2015).
[11] G Bian, T R Chang, R Sankar, S Y Xu, H Zheng et al. Topological nodal-line fermions in spin-orbit metal PbTaSe2. Nat Commun, 7, 10556(2016).
[12] G Bian, T R Chang, H Zheng, S Velury, S Y Xu et al. Drumhead surface states and topological nodal-line fermions in TlTaSe2. Phys Rev B, 93, 121113(2016).
[14] R Yu, H M Weng, Z Fang, X Dai, X Hu. Topological node-line semimetal and dirac semimetal state in antiperovskite Cu3PdN. Phys Rev Lett, 115, 036807(2015).
[15] Y Wu, L L Wang, E Mun, D D Johnson, D X Mou et al. Dirac node arcs in PtSn4. Nat Phys, 12, 667-671(2016).
[16] T R Chang, I Pletikosic, T Kong, G Bian, A Huang et al. Realization of a type-Ⅱ nodal-line semimetal in Mg3Bi2. Adv Sci, 6, 1800897(2019).
[17] J Mao, H T Zhu, Z W Ding, Z H Liu, G A Gamage et al. High thermoelectric cooling performance of n-type Mg3Bi2-based materials. Science, 365, 495-498(2019).
[18] T Zhou, X G Zhu, M Y Tong, Y Zhang, X B Luo et al. Experimental evidence of topological surface states in Mg3Bi2 films grown by molecular beam epitaxy. Chin Phys Lett, 36, 117303(2019).
[19] C M Tu, Y C Chen, P Huang, P Y Chuang, M Y Lin et al. Helicity-dependent terahertz emission spectroscopy of topological insulator Sb2Te3 thin films. Phys Rev B, 96, 195407(2017).
[20] X M Zhang, L Jin, X F Dai, G D Liu. Topological Type-Ⅱ nodal line semimetal and dirac semimetal state in stable kagome compound Mg3Bi2. J Phys Chem Lett, 8, 4814-4819(2017).
[21] J Sánchez-Barriga, E Golias, A Varykhalov, J Braun, L V Yashina et al. Ultrafast spin-polarization control of Dirac fermions in topological insulators. Phys Rev B, 93, 155426(2016).
[22] J Maysonnave, S Huppert, F Wang, S Maero, C Berger et al. Terahertz generation by dynamical photon drag effect in graphene excited by femtosecond optical pulses. Nano Lett, 14, 5797-5802(2014).
[23] H Plank, L E Golub, S Bauer, V V Bel'kov, T Herrmann et al. Photon drag effect in (Bi1-xSbx)2Te3 three-dimensional topological insulators. Phys Rev B, 93, 125434(2016).
[24] L G Zhu, B Kubera, K Fai Mak, J Shan. Effect of surface states on terahertz emission from the Bi2Se3 surface. Sci Rep, 5, 10308(2015).
[25] K X Zhang, Y Z Zhang, X C Wang, T M Yan, Y H Jiang. Continuum electron giving birth to terahertz emission. Photonics Res, 8, 760-767(2020).
[26] D A Bas, R A Muniz, S Babakiray, D Lederman, J E Sipe et al. Photocurrents in Bi2Se3: bulk versus surface, and injection versus shift currents. Opt Express, 24, 23583(2016).
[27] B C Park, T H Kim, K I Sim, B Y Kang, J W Kim et al. Terahertz single conductance quantum and topological phase transitions in topological insulator Bi2Se3 ultrathin films. Nat Commun, 6, 6552(2015).
[28] L He, F X Xiu, X X Yu, M Teague, W J Jiang et al. Surface-dominated conduction in a 6 nm thick Bi2Se3 thin film. Nano Lett, 12, 1486-1490(2012).
[29] Y Pan, Q Z Wang, A L Yeats, T Pillsbury, T C Flanagan et al. Helicity dependent photocurrent in electrically gated (Bi1-xSbx)2Te3 thin films. Nat Commun, 8, 1037(2017).
[30] S Y Hamh, S H Park, S K Jerng, J H Jeon, S H Chun et al. Helicity-dependent photocurrent in a Bi2Se3 thin film probed by terahertz emission spectroscopy. Phys Rev B, 94, 161405(2016).
[31] T Kampfrath, M Battiato, P Maldonado, G Eilers, J Nötzold et al. Terahertz spin current pulses controlled by magnetic heterostructures. Nat Nanotechnol, 8, 256-260(2013).
[32] Y Kinoshita, N Kida, T Miyamoto, M Kanou, T Sasagawa et al. Terahertz radiation by subpicosecond spin-polarized photocurrent originating from Dirac electrons in a Rashba-type polar semiconductor. Phys Rev B, 97, 161104(2018).
[33] Y Wu, M Elyasi, X P Qiu, M J Chen, Y Liu et al. High-performance THz emitters based on ferromagnetic/nonmagnetic heterostructures. Adv Mater, 29, 1603031(2017).
[34] Y M Bahk, G Ramakrishnan, J Choi, H Song, G Choi et al. Plasmon enhanced terahertz emission from single layer graphene. ACS Nano, 8, 9089-9096(2014).
[35] N Sirica, R I Tobey, L X Zhao, G F Chen, B Xu et al. Tracking ultrafast photocurrents in the Weyl semimetal TaAs using THz emission spectroscopy. Phys Rev Lett, 122, 197401(2019).
[36] M J Chen, Y Wu, Y Liu, K Lee, X P Qiu et al. Current-enhanced broadband THz emission from spintronic devices. Adv Opt Mater, 7, 1801608(2019).
[37] J E Moore, J Orenstein. Confinement-induced berry phase and helicity-dependent photocurrents. Phys Rev Lett, 105, 026805(2010).
[38] J P Dong, K P Gradwohl, Y D Xu, T Wang, B B Zhang et al. Terahertz emission from layered GaTe crystal due to surface lattice reorganization and in-plane noncubic mobility anisotropy. Photonics Res, 7, 518-525(2019).
[40] Y Z Hu, T Jiang, J H Zhou, H Hao, H Sun et al. Ultrafast terahertz frequency and phase tuning by all-optical molecularization of metasurfaces. Adv Optical Mater, 7, 1901050(2019).
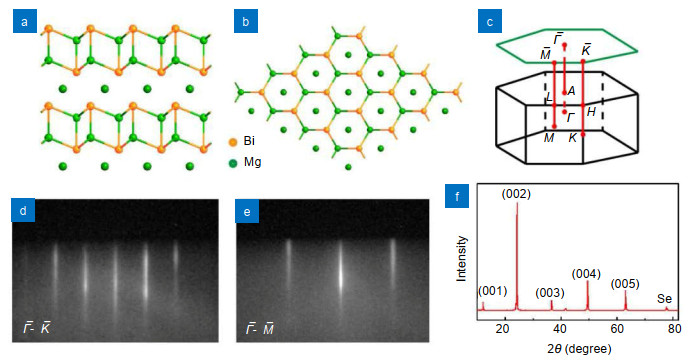
Set citation alerts for the article
Please enter your email address