
- High Power Laser Science and Engineering
- Vol. 2, Issue 3, 03000e26 (2014)
Abstract
1. Introduction
Laser ablation is an efficient removal of a material from its surface and near-surface region irradiated by an intense laser beam at a fluence above the single-shot ablation threshold[ excimer lasers (
[
[
)[
The EUV sources utilized to induce material erosion emit at both low peak power and high peak power. In principle, this results in different modes of material removal[
Quite a different situation is expected if a high-peak-power source delivers a single high-energy pulse to the material. The sample is then exposed to a high local dose of radiation (given by the energy content of the pulse and the absorption length of the radiation in the irradiated material) in a short period of time (given by the pulse duration), i.e., a very high dose rate. This means that a large number of events that cause radiation-induced structural decomposition (i.e., organic polymer chain scissions, etc.) occur almost simultaneously in a relatively thick layer of irradiated material. Since a portion of the radiation energy absorbed in the material will be thermalized, the sudden heating of the layer, which is also heavily chemically altered by the radiation, must be taken into account when the fluence is above the single-shot ablation threshold. The overheated, fragmented region of the irradiated material represents a new phase, which tends to blow off into the vacuum. These particular processes, as well as specific features of short-wavelength ablation with respect to ablation induced by conventional, long-wavelength (UV–Vis–IR) sources, together with their potential applications in micro(nano)structuring, represent a main goal of our research program.
Sign up for High Power Laser Science and Engineering TOC. Get the latest issue of High Power Laser Science and Engineering delivered right to you!Sign up now
In this contribution, a comparison of ablation results obtained in poly(1,4-phenylene ether ether-sulfone) PPEES at 13.5 nm and 46.9 nm is presented.
2. Materials and methods
The ablation experiment has been performed at LLG using poly(methyl methacrylate) (PMMA) as a reference material and then, with the same setup, on a sample material, i.e., PPEES. The material chosen as the target to be tested is PPEES[ electrons, in contrast to the aliphatic molecules of PMMA.
The experimental system used in the EUV irradiation experiments is shown in Figure , repetition rate 5 Hz (typical) and 12 Hz (maximum), capillary lifetime
pulses, current
. The capillary is an
(inner diameter: 3.2 mm, length: 210 mm), Ar-filled (50 Pa). A spherical multilayer Sc/Si mirror with a focal length of 0.25 m was used to focus the CDL beam. The mirror was designed and manufactured to have a maximum reflectivity of 46.9 nm radiation for a
angle between the incident and reflected beams[
The second experimental system, built and operated at LLG, is based on a driving Nd:YAG laser (wavelength 1064 nm, pulse length 7 ns, jitter 1 ns, divergence
0.5 mrad – Gaussian profile at far field, pulse energy stability
1% for 90% of pulses, maximum pulse energy 1.2 J, i.e., twice that used by Barkusky
at 13.5 nm, 2% bw.
This second wavelength is particularly promising because it allows one to modify smaller areas of the material, which is an advantage for many applications (i.e., lithography, nanostructuring).
Typical ablation tests were performed in the tight focus, varying the number of shots. Procedures making it possible to find the focus (i.e., a measurement of caustics, scan) to determine the EUV energy distribution in the focus and to obtain other irradiation characteristics have been explained elsewhere[
The caustics (Figures
3. Results
PMMA was efficiently ablated by 13.5 nm radiation[
This can be explained with respect to our previous positive PPEES ablation results obtained at 46.9 nm (Figures






Therefore, there is at least one order of magnitude higher energy density in the PPEES near-surface region when irradiated at 46.9 nm than in the case of irradiation of the material at 13.5 nm at the same surface energy density. Two conclusions follow from this fact:
The differences in the efficiency of the process explained thanks to the differences in the ablation thresholds and in the efficiency of the radiolytic process mentioned above explain why no ablation was registered at 13.5 nm for fluences achieving a relatively high value, i.e., . It also makes reasonable the finding of ablation craters on the PPEES surface illuminated by 46.9 nm at a lower fluence, i.e., around
.
These considerations provide evidence of the importance of the chemical structure of the material. Indeed, considering just the scaling law related to the optical systems used in this experimental campaign, results at a lower fluence would have been expected. Indeed, the resolution is proportional to the wavelength:








Repeating the experiment on PPEES, which presents a higher radiation stability, is interesting because use of a better resistor would provide an improvement in the industrial application of EUV ablation processes. It guarantees a longer lifetime of such systems before damage.
For this reason, in the next phase of the project, we are going to increase the output energy of the LLG source and focus its EUV emission onto a smaller spot to exceed the EUV ablation threshold of PPEES at 13.5 nm and to evaluate the required fluence experimentally.
However, in future industrial EUV polymer ablation, the wavelength-dependent ablation depth is the guiding parameter; therefore multiple EUV wavelengths may be required, necessitating the use of different elements (gas or solid).
4. Conclusions
In this contribution, we have demonstrated the possibility of creating well-developed ablation craters in PPEES exposed to focused 46.9 nm CDL radiation. However, the more penetrating 13.5 nm radiation (its attenuation length in PPEES exceeds ) was not able to ablate PPEES, although PMMA was ablated quite effectively under these irradiation conditions. The higher radiation stability of PPEES is expected because of the presence of
electrons in the PPEES molecule, in contrast to the less radiation resistant polymer structures (PMMA). The increased attenuation length at the shorter wavelength is likely responsible for the observed difference in the response of PPEES to radiation emitted by the CDL source (
) and the LLG source (
).
References
[1] C. Phipps. Laser Ablation and its Applications(2006).
[2] D. Bäuerle. Laser Processing and Chemistry(2000).
[3] T. Lippert. Plasma Process. Polym., 2, 525(2005).
[4] M. Lapczyna, M. Stuke. Appl. Phys. A, 66, 473(1998).
[5] D. Riedel, M. Claude Castex. Appl. Phys. A, 69, 375(1999).
[6] B. R. Benware, C. D. Macchietto, C. H. Moreno, J. J. Rocca. Phys. Rev. Lett., 81, 5804(1998).
[7] S. Kranzusch, K. Mann. Opt. Commun., 200, 223(2001).
[12] M. J. Bowden, J. H. O’Donnell. Dev. Polym. Degrad., 6, 21(1985).
[13] B. W. Yates, D. M. Shinozaki. J. Mater. Res., 7, 520(1992).
[14] F. Iacona, G. Marletta. Nucl. Instrum. Meth. Phys. Res. B, 166–7, 676(2000).
[15] S. Heinbuch, M. Grisham, D. Martz, J. J. Rocca. Opt. Express, 13, 4050(2005).
[16] L. Vyvsín, T. Burian, J. Chalupský, M. Grisham, V. Hájková, S. Heinbuch, K. Jakubczak, D. Martz, T. Mocek, P. Pira, J. Polan, J. J. Rocca, B. Rus, J. Sobota, L. Juha. Proc. SPIE, 7361, 73610O(2009).
[17] F. Barkusky, C. Peth, A. Bayer, K. Mann. J. Appl. Phys., 101, 124908(2007).
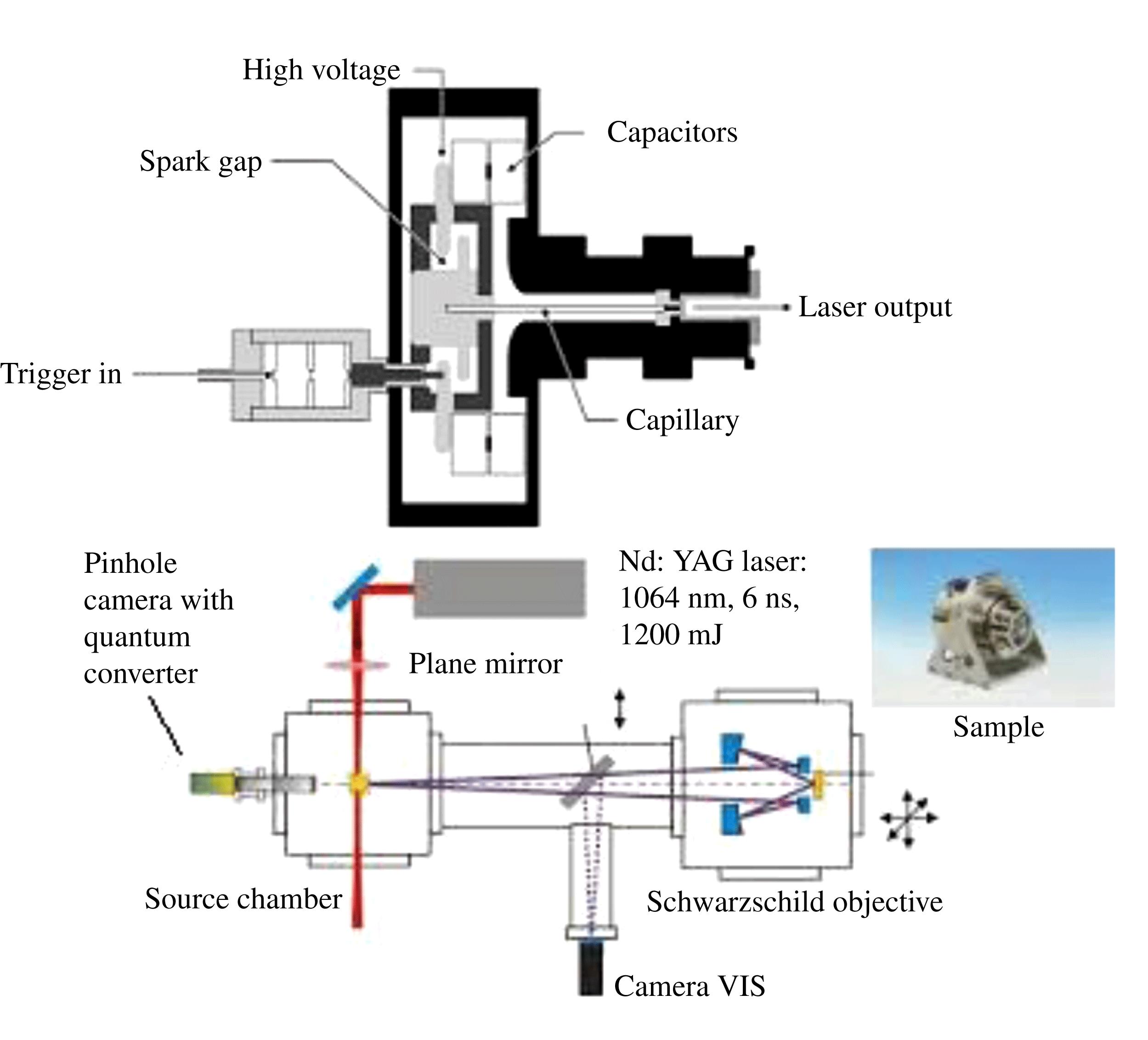
Set citation alerts for the article
Please enter your email address