
- Journal of Semiconductors
- Vol. 41, Issue 1, 010301 (2020)
Abstract
1. What is quantum cascade laser?
Quantum cascade lasers (QCLs) are a rising type of semiconductor lasers and characterized by unique operating principle in comparison with its predecessors, i.e. diode lasers. The most outstanding characteristic of QCLs should be the extraordinary ability of wavelength coverage, theoretically from 3 far to 300 μm, even without the necessity of changing elementary gain materials. Similar to the conventional diode lasers, QCLs basically comprise substrate, low-refractive index lower & upper waveguide cladding layers, and gain region which is multi-layered and quite thicker. We call QCLs the intraband lasers in marked contrast to interband laser which diode lasers belong to. The “intraband” means that carriers driven by external electric field flow directionally in one type of energy band, in practice electrons in conduction band without the interplay of lower valance band. The key strategy to accomplish this task is the utilization of n-doped quantum well and quantum tunneling, which breaks continuous conduction band into a series of semi-discrete subbands in K space and form a string of energy levels spacing from several meV to hundreds of meV due to quantum size confinement effect in quantum well. So the functional elements of QCLs are quantum wells and barriers. Due to the nanoscale barriers, quantum wells are strongly intercoupled resulting in delocalized subband wavefunctions, which is beneficial for efficient electron transition between overlapped states. After applying a certain external electric field the situation of subbands distribution changes a bit, i.e. a series of energy steps or named ladder formed due to added inclined electric potential field. Following the direction of electric field electrons jumps downstream from one subband to another, accompanied by emission or absorption of photons or phonons. The underlying operating mechanism of QCLs is basically a four-level system illustrated in Fig. 1(a), reminiscent of gas or dye lasers, where electrons are injected from level 2’ to level 1’ by resonant tunneling or phonon-assisted scattering, followed by phonon or photon-assisted scattering process from level 1’ to level 2 (in the downstream quantum well) which contributes to spontaneous and stimulated emission of photons of certain frequency, and ended with fast depletion of electrons from level 2 to level 1 in the downstream quantum well (refer to Fig. 1(b)). In fact the prototype in 1970s[
Figure 1.(Color online) (a) Basic four-level system for intersubband lasers and (b) fast LO-phonon scattering process between and in subbands.
2. Milestones and state of the art performance
Since the advent of first prototype of QCLs in 1994[
Figure 2.(Color online) (a) Typical mid infrared QCL structure and (b) subband diagram of gain region under applied electric field.
First QCL was based on strain-compensated InGaAs/InAlAs multi coupled quantum wells grow by MBE and gain region design of diagonal transition plus single phonon resonance extraction (Fig. 3(a)). This device worked up to 90 K at 4.2 μm with peak power in excess of 8 mW. The following was another lattice-matched 8 μm QCL[
Figure 3.(Color online) Representative evolution roadmaps for (a) multi quantum wells design (Refs. [
Aiming at RT CW operation the following refining strategies relating to laser active region includes two main directions, which both concentrate on high-efficiency carrier transport trilogy, i.e. high-efficiency injection, photon emission and depletion. One is to add one or more LO-phonon energy spaced quantum wells after single phonon resonance extraction level for multi coupled quantum wells design, forming so-called double or multi phonon resonance extraction scheme. The effect is obvious: long effective lifetime of lower lasing level restricted by bottleneck effect of resonant tunneling extraction will be lowered to 0.1 ps authentically, while maintains high injection efficiency for single upper lasing level. This modification led to the first RT CW operation of QCLs working up to 312 K with 17 mW output power at 292 K at an emission wavelength of 9.1 μm in 2002[
Driven by the vast and growing applications such as remote sensing, metrology and infrared countermeasures, a variety of modified gain region designs were proposed and implemented successively, bringing high power, high temperature QCLs with extraordinary wavelength coverage into reality: RT CW power of QCLs operating around 5 μm bursts to 5.1 W with wall plug efficiency (WPE) above 21% owing to a shallow well and tall barrier core design in 2011[
3. Where is the limitation?
All the above impressive performance are fundamentally ascribed to a combination of delicate energy band engineering of the laser active region, a refined material growth ensuring repeatability of multi gain stages during a long run of growth, and advanced processing solutions like buried SI-InP heterostructure waveguide for better dissipation of huge active core heat and lower waveguide loss especially for the long wave case. However, beyond the “sweet spot” from 4 μm to 10 μm for QCLs operation, laser performance degrades dramatically. For instance QCLs operating down to 3 μm has a CW power of only several mW due to limited conduction band offset and strong inter-valley scattering[
An ultimate approach aiming at improving QCLs performance radically is to introduce quantum dots in the active region (Fig. 4) utilizing so-called “phonon-bottleneck” mechanism: the zero-dimensional quantization in a quantum dot will break the continuous inplane subbband dispersion curve (especially lower lasing level) into scattered eigenstates, which eliminates electronic states within the energy range of two times of LO-phonon energy at the energy level of upper lasing level and hence suppresses both LO-phonon related relaxation and undesired dephasing scattering of electrons in upper lasing level. This approach was proposed as soon as the invention of quantum well cascade lasers[
Figure 4.(Color online) Schematic of (a) a quantum dot cascade laser and (b) energy band structures of quantum wells and quantum dots-based active region.
References
[1] R Kazarinov, R A Suris. Possibility of the amplification of electromagnetic waves in a semiconductor with a superlattice. Sov Phys Semicond, 5, 707(1971).
[2] J Faist, F Capasso, D L Sivco et al. Quantum cascade laser. Science, 264, 553(1994).
[3] G Scamarcio, F Capasso, C Sirtori et al. High-power infrared (8-micrometer wavelength) superlattice lasers. Science, 276, 773(1997).
[4] R Kohler, A Tredicucci, F Beltram et al. Terahertz semiconductor heterostructure laser. Nature, 417, 156(2002).
[5] M Beck, D Hofstetter, T Aellen et al. Continuous wave operation of a mid-Infrared semiconductor laser at room temperature. Science, 295, 301(2002).
[6] M Rochat, D Hofstetter, M Beck et al. Long-wavelength 16 mm, room-temperature, single-frequency quantum-cascade lasers based on a bound-to-continuum transition. Appl Phys Lett, 79, 4271(2001).
[7] G Scalari, L Ajili, J Faist et al. Far-infrared (87
[8] Y Bai, N Bandyopadhyay, S Tsao et al. Room temperature quantum cascade lasers with 27% wall plug efficiency. Appl Phys Lett, 98, 181102(2011).
[9] A Lyakh, R Maulini, A Tsekoun et al. Multiwatt long wavelength quantum cascade lasers based on high strain composition with 70% injection efficiency. Opt Express, 20, 24272(2012).
[10] F Xie, C Caneau, H P Leblanc et al. Watt-level room temperature continuous-wave operation of quantum cascade lasers with λ >10
[11] S Fathololoumi, E Dupont, C E I Chan et al. Terahertz quantum cascade lasers operating up to ~ 200 K with optimized oscillator strength and improved injection tunneling. Opt Express, 20, 3866(2012).
[12] L Bosco, M Franckie, G Scalari et al. Thermoelectrically cooled THz quantum cascade laser operating up to 210 K. Appl Phys Lett, 115, 010601(2019).
[13] M A Belkini, F Capasso, A Belyanin et al. Terahertz quantum-cascade-laser source based on intracavity difference-frequency generation. Nat Photonics, 1, 288(2007).
[14] Q Y Lu, N Bandyopadhyay, S Slivken et al. Continuous operation of a monolithic semiconductor terahertz source at room temperature. Appl Phys Lett, 104, 221105(2014).
[15] A Hugi, G Villares, B Blaser et al. Mid-infrared frequency comb based on a quantum cascade laser. Nature, 492, 229(2012).
[16] Q Lu, D Wu, S Slivken et al. High efficiency quantum cascade laser frequency comb. Sci Rep, 7, 43806(2017).
[17] D Kazakov, M Piccardo, Y Wang et al. Self-starting harmonic frequency comb generation in a quantum cascade laser. Nat Photonics, 11, 789(2017).
[18] N Bandyopadhyay, Y Bai, S Tsao et al. Room temperature continuous wave operation of
[19] S Niu, J Liu, F Cheng et al. 14
[20] M Bahriz, G Lollia, A N Baranov et al. High temperature operation of far infrared (λ ≈ 20
[21] E Bellotti, K Driscoll, T D Moustakas et al. Monte Carlo study of GaN versus GaAs terahertz quantum cascade structures. Appl Phys Lett, 92, 101112(2008).
[22] N S Wingreen, C A Stafford. Quantum-dot cascade laser: proposal for an ultralow-threshold semiconductor laser. IEEE J Quantum Electron, 33, 1170(1997).
[23] B A Burnett, B S Williams. Density matrix model for polarons in a terahertz quantum dot cascade laser. Phys Rev B, 90, 155309(2014).
[24] N Zhuo, J Zhang, F Wang et al. Room temperature continuous wave quantum dot cascade laser emitting at 7.2
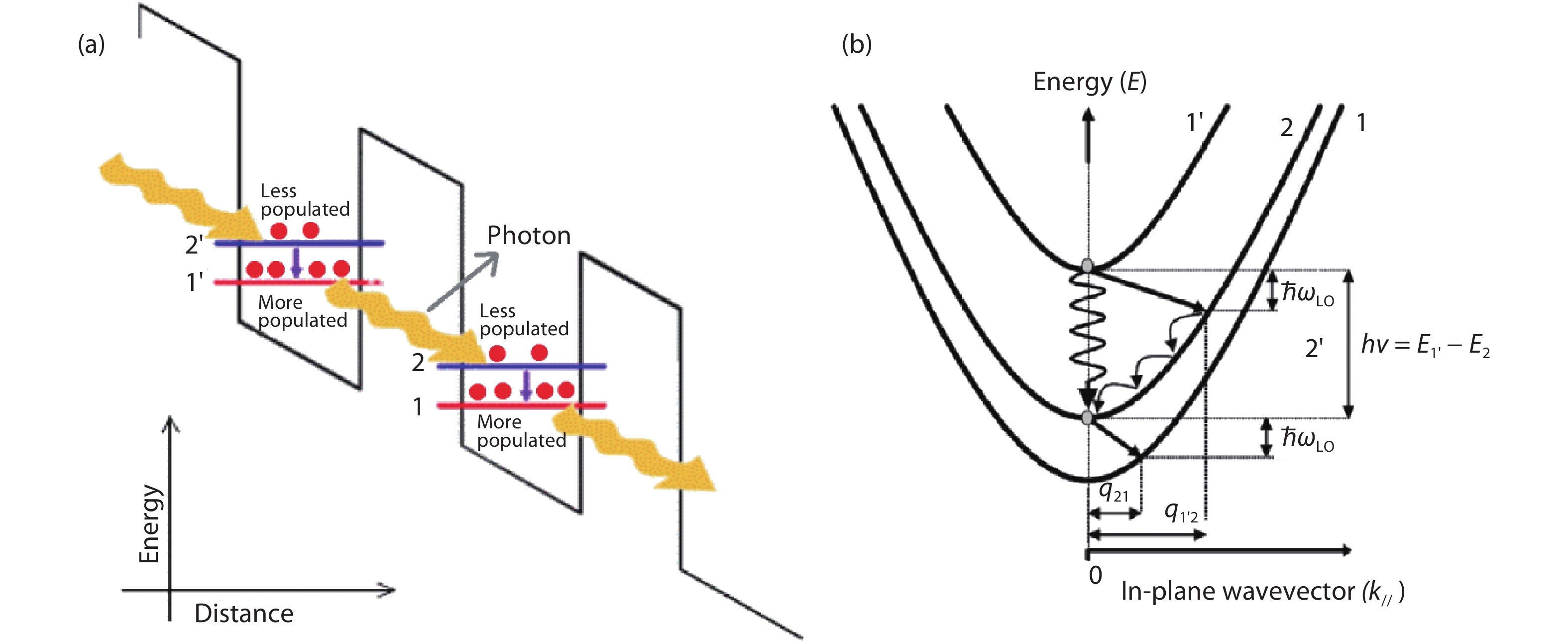
Set citation alerts for the article
Please enter your email address