
- Photonics Research
- Vol. 10, Issue 6, 1417 (2022)
Abstract
1. INTRODUCTION
Single-photon source is an essential element in photonic implementations of quantum technologies, which ideally emits single photons in a pure, deterministic, and indistinguishable manner that can ensure several potential applications such as fundamental investigations of the quantum nature of light [1,2], security communication [3,4], exponentially enhanced calculation [5], and high accuracy measurement [6]. To make single-photon emission more applicable for wide-spread deployment in quantum techniques, two different avenues have been paved, which are based on single emitters [7] and spontaneous non-linear parametric processes, respectively. The first one is capable of emitting single photons deterministically, but it needs complex fabrication processes and is difficult to operate at room temperature. The second pathway is the heralded single-photon source (HSPS) [8], which is based on correlated photon pairs generated from spontaneous parametric downconversion (SPDC) or spontaneous four-wave mixing. The detection of one of the photons in the photon pair, the heralding photon, indicates the existence of its twin photon, the heralded photon. Such HSPSs are experimentally convenient, emission wavelength flexible, and most importantly, highly indistinguishable. However, the spontaneous non-linear parametric processes bring inevitable probabilistic property [9]. In particular, to achieve higher single-photon purity, the HSPS system has to operate at low pumping power level, which limits the heralded single-photon (HSP) rate and hinders the practical application of HSPSs.
To break this trade-off between the HSP rate and the single-photon purity and to develop HSPSs toward a higher efficiency and deterministic manner, one promising way is to multiplex a set of HSPSs (different modes) into one common output [8,10,11]. In such a multiplexing system, the detection of a heralding photon indicates the presence of a heralded photon at this certain mode. Many multiplexing schemes have been demonstrated, with modes in different degrees of freedom including space [12–17], time [17–20], frequency [21–23], and orbital angular momentum (OAM) [24]. Among these schemes, multiplexing HSPSs in the frequency domain (i.e., spectral multiplexing) attract great interest for their promising scalability as well as fixed losses and requirement of resources. There have been demonstrated spectral multiplexing HSPSs at wavelengths of
In this paper, we propose a chip-scale HSPS on lithium niobate on insulator (LNOI) at 1.5 μm telecom-band based on spectral multiplexing and feed-forward control, as well as demonstrate a proof-of-principle experiment with discrete fiber-based components. In our proposal, all of the integrated components and those couplings among them can be realized by readily available technologies in LNOI-based integrated photonics. In the proof-of-principle demonstration, broadband correlated photon pairs are generated by the cascaded second-order harmonics generation (SHG) and SPDC processes in an integrated periodically poled lithium niobate (PPLN) waveguide module [30]. Three spectral modes within the idler spectrum are defined as the heralding spectral modes while different active feed-forward spectral manipulations are applied on the signal photon side when a corresponding heralding photon is detected in a spectral mode-resolved way. Our experimental results show a breakthrough of trade-off between the HSP rate and the single-photon purity. With continuous-wave (CW) laser pumping and three modes multiplexed, the measured HSP rate is enhanced by near threefold toward 3.1 kHz at low pumping power level while keeping an ultra-low
Sign up for Photonics Research TOC. Get the latest issue of Photonics Research delivered right to you!Sign up now
2. PRINCIPLES AND RESULTS
A. Principle of Future On-Chip Spectrally Multiplexed Single-Photon Source
Crystalline lithium niobate has been widely used in research of optical quantum technologies for its high non-linearity, high electro-optic coefficients, and broadband transmission window [33]. In recent years, the development and commercialization of technologies in manufacturing and fabricating LNOI has made this platform a promising candidate in realizing complex quantum photonic circuit [34,35]. Here, we propose an integrated scheme of spectrally multiplexed HSPS based on an LNOI platform, which includes a pump laser module, photon-pair generation module, filtering and photon detecting module, and feed-forward and frequency shifting module, as illustrated in Fig. 1(a). In particular, the pump laser module injects light at 1.5 μm into the photon-pair generation module to induce non-linear processes. The pump laser module can be an InP laser transferred and placed onto an unpatterned insulator layer, which can be realized by pick-and-place tools [36,37]. The photon-pairs generation module includes a PPLN waveguide on LNOI [38–40] in which enhanced cascaded SHG and SPDC [41,42] processes occur and then correlated photon pairs are generated, as shown in Fig. 1(b). Photonic wire bonding can be used for low-loss coupling from the pump laser to the PPLN waveguide [43–45]. This method can also be employed to couple the generated signal and idler photons into the following filtering and photon detecting module. In the filtering and photon detecting module, signal photons in a certain frequency band are selected by a demultiplexer and then delayed by a section of on-chip delay line, while their idler twins are selected with finer frequency grids and finally coupled into on-chip superconducting nanowire single-photon detectors (SNSPDs). The on-chip demultiplexer can be realized by arrayed waveguide gratings [46–48] or cascaded Mach–Zehnder interferometers [49] on LNOI. The optical true delay lines on LNOI with low propagation losses [50] and LNOI waveguide-integrated SNSPDs [51,52] required in the filtering and photon detecting module are both in the reach of recent technology. Detection events of idler photons generate heralding electronic signals, which are then sent to an integrated logic circuit in the feed-forward and frequency shifting module via bonded wires [53]. At the same time, the signal photons are coupled into an electro-optic phase modulator (EOM) on LNOI [54–56]. Driven by the frequency shifting signals output from the integrated logic circuit, the EOM converts the frequency of signal photons from different spectral modes, as shown in Fig. 1(c). Detection events of idler photons from different frequency channels lead to electronic signals with different magnitudes of frequency shifting for signal photons, which guarantees that all the signal photons are finally converted into a common spectral mode. After the frequencyshifting operation, the signal photons are coupled out for applications.
Figure 1.Principles of chip-scale LNOI-based spectrally multiplexed HSPS. (a) A view of future chip-scale LNOI-based spectrally multiplexed HSPS, in which all basic components are integrated on an LNOI photonic chip including pump laser module, (I) photon-pairs generation module, (II) filtering and detecting module, and (III) feed-forward and frequency shifting module. (b) Energy conservation graph of SHG and SPDC. (c) Illustration of multiplexing in frequency domain. Photon-pairs generation module (I) generates broadband correlated signal and idler photons through cascaded SHG and SPDC processes. Idler photons are filtered into different spectral modes and detected by SNSPDs in the filtering and detecting module (II). Detection signals are sent to the logic circuit. The logic circuit sends frequency shifting signal to the feed-forward and frequency shifting module (III), where signal photons are shifted into the common spectral mode. LNOI, lithium niobate on insulator; PPLN, periodically poled lithium niobate; LN, lithium niobate; EOM, electro-optic phase modulator; SNSPD, superconducting nanowire single-photon detector; Freq. Shif. Sig., frequency shifting signals; SHG, second-order harmonics generation; SPDC, spontaneous parametric downconversion.
B. Proof-of-Principle Experimental Setup
The viability of the concept illustrated in Fig. 1 is demonstrated in a proof-of-principle experiment with discrete fiber-based components. Figure 2 shows the experimental setup. In this scheme, broadband photon pairs are generated by cascaded SHG and Type-0 SPDC processes in a fiber-connected PPLN waveguide. Under this configuration of non-linear processes, the wavelengths of pumping photons, signal photons, and heralding photons are all at 1.5 μm telecom-band, which is compatible with optical fiber telecommunication systems. After exiting from the PPLN waveguide, correlated signal photons and heralding photons with a bandwidth of
Figure 2.Experimental setup for spectrally multiplexed HSPS. PPLN, periodically poled lithium niobate; DWDM, dense wavelength-divided multiplexer; EOM, electro-optic phase modulator; TNF, tunable narrowband filter; BS, beam splitter; PC, polarization controller; TDC, time-to-digit convertor; SNSPD, superconducting nanowire single-photon detector.
We verify the spectral property of the heralded signal photons with and without applying the frequency shifting signal, respectively. Here, signal photons are selected in the frequency domain by varying the filtering window of a tunable narrowband filter (TNF) which has a bandwidth of 12.5 GHz. The coincidence events between signal photons and heralding photons are counted by the coincidence logic circuit. The results are shown in Fig. 3. In Fig. 3(a), without applying frequency shifting, it is obvious that the spectra of signal photons are separated into three spectral modes,
Figure 3.Coincidence count rates without and with frequency shifting. (a) and (b) are the measured coincidence count rates among three individual spectral modes without and with spectral multiplexing, respectively, under the CW pumping power of 4 mW. The horizontal axis corresponds to the relative frequency difference with respect to
C. Brightness and Coincidences-to-Accidentals Ratio
We characterize the source brightness, i.e., HSP rate, with experimental setup running in both multiplexing enabled and disabled cases, when CW lasers with different pump powers are applied, as shown in Fig. 4(a). Here, the HSP rate is defined as the coincidence count rate measured in the experiment without the correction for detector efficiency. The multiplexed HSP rate is measured by fixing the TNF at the center frequency of
Figure 4.Experimental results from multiplexing of three spectral modes. Blue diamonds are multiplexed source, and red rectangles, orange circles, and yellow triangles represent individual spectral modes of
In Fig. 4(a), it can be seen that the multiplexed source has an HSP rate enhanced by a factor of
The coincidences-to-accidentals ratio (CAR) is a powerful tool to estimate how many undesired noise photons are generated together with the genuine correlated photon pairs. It can be defined as the ratio of the coincidence count rate between signal and heralding photons to the accidental count rate. We measure the CAR versus HSP rate for the multiplexed source and three individual modes. As shown in Fig. 4(b), the CAR of the multiplexed source is higher than those of individual modes. The CAR decreases at a higher HSP rate, since the main source of noise is Raman scattering photons and multipair events [30]. In our demonstration, a high-performance multiplexing HSPS is obtained with the CAR higher than 2000 at the HSP rate of 4 kHz. This value of multiplexed HSP rate also shows an enhancement of
D. Single-Photon Purity
We obtain the single-photon purity of our spectrally multiplexed HSPS by measuring the second-order autocorrelation function
E. Joint Spectral Intensity and Hong–Ou–Mandel Interference
We study the spectral property of our proposed multiplexed HSPS by analyzing the JSI of photon pairs as well as HOM interference between the multiplexed HSPS and a weak coherence single-photon source. The JSI is given by the mode square of joint spectral amplitude [58] which can be measured by signal–idler photon coincidences versus
Pumping the PPLN waveguide in Fig. 2 with pulsed laser, we measure the JSI (see Appendix D). Figures 5(a)–5(c) show the JSIs of photon pairs directly output from PPLN without filtering, photon pairs with narrowband DWDM inserted into the idler arm when multiplexing is disabled, and photon pairs with multiplexing enabled. As shown in Fig. 5(a), for the broadband nature of the PPLN waveguide, the JSI exhibits a diagonal band with strong frequency correlation. The cross-sectional width
Figure 5.Joint spectrum intensity (JSI) between heralding and signal photons in the
To further characterize the non-classical nature and indistinguishability of our spectrally multiplexed HSPS, we demonstrate an experiment of HOM interference between the multiplexed HSPS and an independent weak coherence single-photon source. HOM interference from independent sources is very important in many applications such as quantum teleportation [25,26] and MDI-QKD [31]. For general photon-pair sources, the individual signal mode or heralding mode does not deliver a non-classical photon-number statistics unless extracting the single photon nature by the heralding procedure [60]. Visibility higher than 50% is used as a criterion to determine whether the non-classicality takes place in HOM interference between photons from independent sources [61]. The weak coherence source not only serves as the quasi-single-photon source for the HOM effect from independent sources, but also, this kind of interference between single photons and photons from a weak coherence source can be used in some areas such as homodyne detection [62] and quantum circuits [63]. Here, the HOM interference experiment is carried out by launching balanced field intensity into two input ports, meaning that the mean photon numbers are equal when two input fields are respectively launched into two input ports of the beam splitter. As shown in Fig. 6, the visibility of twofold coincidences without the heralding procedure is
Figure 6.HOM interference between the multiplexed source and the weak coherence source. Red circles are twofold coincidences measured without the heralding procedure. Blue diamonds are threefold coincidences measured under the condition by the heralding procedure without subtracting the contribution of the multiphoton event. Both twofold and threefold coincidences are fitted by Gaussian functions with red and blue curves and have the visibility of
3. DISCUSSION AND CONCLUSION
In this paper, we propose a chip-scale LNOI-based spectral multiplexing HSPS at telecom-band and demonstrate a proof-of-principle experiment, yielding a significantly enhanced performance using discrete fiber-based components. The enhancement is possible to behave as our theoretical model and perfectly close to the number of multiplexing modes when the faster electronic devices are utilized. Furthermore, the first implementation of HOM interference from independent sources between our multiplexed source and a weak coherence single-photon source shows the evidence of non-classical nature. The results show the viability that large-scale integration of photonic chip HSPS has great potential in realizing a high-performance single-photon source.
In our demonstration, we use a tunable filter as the output filter for HSPS and a fiber-based EOM for frequency shifting, which both introduce relatively high loss. These losses, as well as losses from other fiber-based components, will degrade the photons collection efficiency and heralding efficiency (see details in Appendix F). The use of an integrated platform (see Fig. 1) will address the problem of component and connection losses as well as improve the performance of HSPS significantly.
Furthermore, scalable multiplexing is promising in our proposed integrated scheme if a larger magnitude of frequency shifting is applied. The recently developed integrated lithium niobate EOM has low
We note that a related result of spectrally multiplexed HSPS at 1.5 μm has been implemented by Hiemstra
Summary of Single-Photon Source (SPS) Performance among Previous Demonstrations
Year | Types of SPS | MUX DOF | Modes Num. | Temp. | Rate (kHz) | EF | Refs. | ||
---|---|---|---|---|---|---|---|---|---|
2016 | HSPS | Space | 1550 | 2 | RT | 0.05 | 0.5 | 1.75 | [ |
2016 | HSPS | Time | 1545 | 4 | RT | – | 0.6 | 2 | [ |
2016 | HSPS | Space & Time | 1547 | 8 | RT | – | 0.3 | 2.14 | [ |
2016 | QD | – | – | 8 K | 0.00044 | 196 | – | [ | |
2016 | QD | – | – | 80 K | 0.34 | – | – | [ | |
2017 | HSPS | Freq. | 795 | 3 | RT | 0.06 | 0.3 | [ | |
2018 | HSPS | Freq. | 1280 | 3 | RT | 0.07 | 23 | 2.2 | [ |
2018 | QD | – | – | 4 K | 0.000075 | 60 | – | [ | |
2019 | HSPS | OAM | 1550 | 3 | RT | 0.048 | 1.47 | [ | |
2019 | HSPS | Time | 1590 | 40 | RT | 0.007 | 25.5 | 27.9 | [ |
0.088 | 206 | 18.7 | |||||||
0.269 | 334 | 9.7 | |||||||
2019 | QD | – | – | 1.5 K | 0.025 | 13700 | – | [ | |
2020 | HSPS | Freq. | 1565 | RT | 2.7 | [ | |||
2.7 | |||||||||
MUX, multiplexing; DOF, degree of freedom; Temp., temperature; EF, enhancement factor; RT, room temperature; QD, quantum dot; Freq., frequency. The rate in the table refers to the measured rate of coincidence in the experiment.
APPENDIX A: DETAILS OF THE EXPERIMENTAL SETUP
As described in the main text, the proof-of-principle demonstration of spectrally multiplexed HSPS in discrete fiber-based components is experimentally realized as follows. Spectral mode-resolved detection of heralding photons tells the feed-forward logic about the frequency information while the feed-forward logic then gives the frequency converter, i.e., electro-optic modulator, a corresponding frequency shifting signal. Finally signal photons are shifted into the central spectral mode. Here, the pumping field centered at
APPENDIX B: FREQUENCY SHIFTING SIGNAL
In our demonstration of spectrally multiplexed HSPS, the frequency shifting signal is generated from a series of electronic devices; see Fig.
Figure 7.(a) Experimental setup for generation of frequency shifting signals. AWG, arbitrary waveform generator; Amp., microwave amplifier. (b) Typical electronic signal for frequency shifting. (c) Enhancement of the multiplexed source versus heralding rate. Inset: Heralding efficiency of the multiplexed source versus pump power. Green circles and blue diamonds are measured data while green and blue dashed curves are the result of theoretical calculation. Error bars are estimated using Poisson statistics.
The enhancement versus heralding rate of the multiplexed source system is shown in Fig.
APPENDIX C: SCALABLE MULTIPLEXING
The currently available AWG with 100 GSa/s sampling rate shows a fourfold improvement compared with our scheme, while the
To get a net enhancement after spectral multiplexing, the loss of heralded photons introduced by frequency shifting devices should be compensated by the multiplexing. In detail, before multiplexing, the probability for a click on detector of heralded photons per pulse is [
For space or time multiplexing systems, the additional system loss
APPENDIX D: MEASUREMENT OF JOINT SPECTRAL INTENSITY AND HONG–OU–MANDEL INTERFERENCE
In the scheme of JSI measurement, we add another TNF (OTF-970, Santec) in the heralding arm before the narrowband DWDM (not shown in the main text Fig.
The multiplexing HSPS and a weak coherence source are used to perform the HOM interference. The pulsed laser is synchronized with the photon-pair source with a pulse bandwidth of 6.4 GHz and a repetition rate of 500 MHz. The HOM effect only occurs when two input photons are indistinguishable. Thus, the wavelengths of the two sources are both centered at 1532.03 nm (
APPENDIX E: VISIBILITY OF HONG–OU–MANDEL INTERFERENCE
To understand the result of Fig.
Now, consider the input fields
In our implementation we have balanced input fields, which means that the mean photon numbers for the two input fields are equal, i.e.,
Next, we consider the input fields
Again, in a balanced input with
In HOM interference, the premise for ideal non-classical interference is that the photon participating in the interference is spectrally pure. The imperfection of single-photon spectral purity should be taken into consideration when revealing the non-classical nature of the threefold coincidence. For the imperfect spectral purity, we should take into account the unwanted external broadening mechanism. In our experiment, the bandwidth of the Gaussian pumping pulses is 6.4 GHz. Considering the SHG process and heralding procedure, the heralded signal photons have a broadened external bandwidth of 11.2 GHz, which is a convolution result of pump photon bandwidth and heralding photon bandwidth. Such mismatch between the internal and external bandwidth reduces the spectral purity of the single photon and shows an 81.16% deterioration of visibility [
Figure 8.Threefold coincidences before (green diamonds) and after (blue diamonds) correction. Error bars are estimated using Poisson statistics.
APPENDIX F: LOSSES AND EFFICIENCY
The implementation of the multiplexed HSPS has many fiber-based optical components, which introduce relatively large losses causing inefficient photon collection on both the heralding and heralded sides. We measure the overall component losses and efficiencies of the signal arm and heralding arm in three spectral modes, as shown in Tables
Overall Component Losses of the Signal and Heralding Arms
Components | Losses (dB) |
---|---|
PPLN waveguide output coupling | 2.50 |
DWDM-signal | 1.83 |
DWDM-heralding | 2.21 |
Narrowband DWDM- | 4.58 |
Narrowband DWDM- | 4.58 |
Narrowband DWDM- | 4.60 |
Delay fiber | 0.40 |
EOM | 4.56 |
TNF | 5.30 |
SNSPDs | 1.80–2.20 |
PPLN, periodically poled lithium niobate; DWDM, dense wavelength divided multiplexer; EOM, electro-optic phase modulator; TNF, tunable narrowband filter; SNSPD, superconducting nanowire single-photon detector.
Total Transmission Efficiencies and Collection Efficiencies of the Signal and Heralding Arms
Efficiencies | Value (%) |
---|---|
Transmission efficiency-signal arm | 2.10 |
Transmission efficiency-heralding arm | 7.06 |
Heralding efficiency | 2.15 |
Collection efficiency- | 5.34 |
Collection efficiency- | 4.87 |
Collection efficiency- | 5.43 |
References
[1] C.-K. Hong, Z.-Y. Ou, L. Mandel. Measurement of subpicosecond time intervals between two photons by interference. Phys. Rev. Lett., 59, 2044-2046(1987).
[2] Y.-H. Deng, H. Wang, X. Ding, Z.-C. Duan, J. Qin, M.-C. Chen, Y. He, Y.-M. He, J.-P. Li, Y.-H. Li, L.-C. Peng, E. S. Matekole, T. Byrnes, C. Schneider, M. Kamp, D.-W. Wang, J. P. Dowling, S. Höfling, C.-Y. Lu, M. O. Scully, J.-W. Pan. Quantum interference between light sources separated by 150 million kilometers. Phys. Rev. Lett., 123, 080401(2019).
[3] N. Gisin, G. Ribordy, W. Tittel, H. Zbinden. Quantum cryptography. Rev. Mod. Opt., 74, 145-195(2002).
[4] N. Gisin, R. Thew. Quantum communication. Nat. Photonics, 1, 165-171(2007).
[5] J. L. O’brien. Optical quantum computing. Science, 318, 1567-1570(2007).
[6] V. Giovannetti, S. Lloyd, L. Maccone. Quantum metrology. Phys. Rev. Lett., 96, 010401(2006).
[7] I. Aharonovich, D. Englund, M. Toth. Solid-state single-photon emitters. Nat. Photonics, 10, 631-641(2016).
[8] E. Meyer-Scott, C. Silberhorn, A. Migdall. Single-photon sources: approaching the ideal through multiplexing. Rev. Sci. Instrum., 91, 041101(2020).
[9] A. Christ, C. Silberhorn. Limits on the deterministic creation of pure single-photon states using parametric down-conversion. Phys. Rev. A, 85, 023829(2012).
[10] A. L. Migdall, D. Branning, S. Castelletto. Tailoring single-photon and multiphoton probabilities of a single-photon on-demand source. Phys. Rev. A, 66, 053805(2002).
[11] T. Pittman, B. Jacobs, J. Franson. Single photons on pseudodemand from stored parametric down-conversion. Phys. Rev. A, 66, 042303(2002).
[12] X.-S. Ma, S. Zotter, J. Kofler, T. Jennewein, A. Zeilinger. Experimental generation of single photons via active multiplexing. Phys. Rev. A, 83, 043814(2011).
[13] M. J. Collins, C. Xiong, I. H. Rey, T. D. Vo, J. He, S. Shahnia, C. Reardon, T. F. Krauss, M. Steel, A. S. Clark, B. Eggleton. Integrated spatial multiplexing of heralded single-photon sources. Nat. Commun., 4, 2582(2013).
[14] C. Xiong, T. D. Vo, M. Collins, J. Li, T. Krauss, M. Steel, A. Clark, B. Eggleton. Bidirectional multiplexing of heralded single photons from a silicon chip. Opt. Lett., 38, 5176-5179(2013).
[15] T. Meany, L. A. Ngah, M. J. Collins, A. S. Clark, R. J. Williams, B. J. Eggleton, M. Steel, M. J. Withford, O. Alibart, S. Tanzilli. Hybrid photonic circuit for multiplexed heralded single photons. Laser Photonics Rev., 8, L42-L46(2014).
[16] R. J. Francis-Jones, R. A. Hoggarth, P. J. Mosley. All-fiber multiplexed source of high-purity single photons. Optica, 3, 1270-1273(2016).
[17] G. J. Mendoza, R. Santagati, J. Munns, E. Hemsley, M. Piekarek, E. Martn-López, G. D. Marshall, D. Bonneau, M. G. Thompson, J. L. O’Brien. Active temporal and spatial multiplexing of photons. Optica, 3, 127-132(2016).
[18] F. Kaneda, B. G. Christensen, J. J. Wong, H. S. Park, K. T. McCusker, P. G. Kwiat. Time-multiplexed heralded single-photon source. Optica, 2, 1010-1013(2015).
[19] C. Xiong, X. Zhang, Z. Liu, M. J. Collins, A. Mahendra, L. Helt, M. J. Steel, D.-Y. Choi, C. Chae, P. Leong, B. J. Eggleton. Active temporal multiplexing of indistinguishable heralded single photons. Nat. Commun., 7, 10853(2016).
[20] F. Kaneda, P. G. Kwiat. High-efficiency single-photon generation via large-scale active time multiplexing. Sci. Adv., 5, eaaw8586(2019).
[21] M. G. Puigibert, G. Aguilar, Q. Zhou, F. Marsili, M. Shaw, V. Verma, S. Nam, D. Oblak, W. Tittel. Heralded single photons based on spectral multiplexing and feed-forward control. Phys. Rev. Lett., 119, 083601(2017).
[22] C. Joshi, A. Farsi, S. Clemmen, S. Ramelow, A. L. Gaeta. Frequency multiplexing for quasi-deterministic heralded single-photon sources. Nat. Commun., 9, 847(2018).
[23] T. Hiemstra, T. Parker, P. Humphreys, J. Tiedau, M. Beck, M. Karpiński, B. Smith, A. Eckstein, W. Kolthammer, I. Walmsley. Pure single photons from scalable frequency multiplexing. Phys. Rev. Appl., 14, 014052(2020).
[24] S.-L. Liu, Q. Zhou, Z.-Y. Zhou, S.-K. Liu, Y. Li, Y.-H. Li, C. Yang, Z.-H. Xu, G.-C. Guo, B.-S. Shi. Multiplexing heralded single photons in orbital-angular-momentum space. Phys. Rev. A, 100, 013833(2019).
[25] R. Valivarthi, Q. Zhou, G. H. Aguilar, V. B. Verma, F. Marsili, M. D. Shaw, S. W. Nam, D. Oblak, W. Tittel. Quantum teleportation across a metropolitan fibre network. Nat. Photonics, 10, 676-680(2016).
[26] Q.-C. Sun, Y.-L. Mao, S.-J. Chen, W. Zhang, Y.-F. Jiang, Y.-B. Zhang, W.-J. Zhang, S. Miki, T. Yamashita, H. Terai, X. Jiang, T.-Y. Chen, L.-X. You, X.-F. Chen, Z. Wang, J.-Y. Fan, Q. Zhang, J.-W. Pan. Quantum teleportation with independent sources and prior entanglement distribution over a network. Nat. Photonics, 10, 671-675(2016).
[27] H. Liu, C. Jiang, H.-T. Zhu, M. Zou, Z.-W. Yu, X.-L. Hu, H. Xu, S. Ma, Z. Han, J.-P. Chen, Y. Dai, S.-B. Tang, W. Zhang, H. Li, L. You, Z. Wang, Y. Hua, H. Hu, H. Zhang, F. Zhou, Q. Zhang, X.-B. Wang, T.-Y. Chen, J.-W. Pan. Field test of twin-field quantum key distribution through sending-or-not-sending over 428 km. Phys. Rev. Lett., 126, 250502(2021).
[28] J.-P. Chen, C. Zhang, Y. Liu, C. Jiang, W.-J. Zhang, Z.-Y. Han, S.-Z. Ma, X.-L. Hu, Y.-H. Li, H. Liu, F. Zhou, H.-F. Jiang, T.-Y. Chen, H. Li, L.-X. You, Z. Wang, X.-B. Wang, Q. Zhang, J.-W. Pan. Twin-field quantum key distribution over a 511 km optical fibre linking two distant metropolitan areas. Nat. Photonics, 15, 570-575(2021).
[29] E. Saglamyurek, M. G. Puigibert, Q. Zhou, L. Giner, F. Marsili, V. B. Verma, S. W. Nam, L. Oesterling, D. Nippa, D. Oblak, W. Tittel. A multiplexed light-matter interface for fibre-based quantum networks. Nat. Commun., 7, 11202(2016).
[30] Z. Zhang, C. Yuan, S. Shen, H. Yu, R. Zhang, H. Wang, H. Li, Y. Wang, G. Deng, Z. Wang, L. You, Z. Wang, H. Song, G.-C. Guo, Q. Zhou. High-performance quantum entanglement generation via cascaded second-order nonlinear processes. npj Quantum Inf., 7, 1(2021).
[31] K. Wei, W. Li, H. Tan, Y. Li, H. Min, W.-J. Zhang, H. Li, L. You, Z. Wang, X. Jiang, T.-Y. Chen, S.-K. Liao, C.-Z. Peng, F. Xu, J.-W. Pan. High-speed measurement-device-independent quantum key distribution with integrated silicon photonics. Phys. Rev. X, 10, 031030(2020).
[32] E. Knill, R. Laflamme, G. J. Milburn. A scheme for efficient quantum computation with linear optics. Nature, 409, 46-52(2001).
[33] J. Lin, F. Bo, Y. Cheng, J. Xu. Advances in on-chip photonic devices based on lithium niobate on insulator. Photon. Res., 8, 1910-1936(2020).
[34] S. Saravi, T. Pertsch, F. Setzpfandt. Lithium niobate on insulator: an emerging platform for integrated quantum photonics. Adv. Opt. Mater., 9, 2100789(2021).
[35] D. Zhu, L. Shao, M. Yu, R. Cheng, B. Desiatov, C. Xin, Y. Hu, J. Holzgrafe, S. Ghosh, A. Shams-Ansari, E. Puma, N. Sinclair, C. Reimer, M. Zhang, M. Lončar. Integrated photonics on thin-film lithium niobate. Adv. Opt. Photonics, 13, 242-352(2021).
[36] S. Aghaeimeibodi, B. Desiatov, J.-H. Kim, C.-M. Lee, M. A. Buyukkaya, A. Karasahin, C. J. Richardson, R. P. Leavitt, M. Lončar, E. Waks. Integration of quantum dots with lithium niobate photonics. Appl. Phys. Lett., 113, 221102(2018).
[37] J. Zhang, G. Muliuk, J. Juvert, S. Kumari, J. Goyvaerts, B. Haq, C. Op de Beeck, B. Kuyken, G. Morthier, D. Van Thourhout, A. Gocalinska, J. O’Callaghan, E. Pelucchi, K. Thomas, B. Corbett, A. J. Trindade, G. Roelkens. III-V-on-Si photonic integrated circuits realized using micro-transfer-printing. APL Photonics, 4, 110803(2019).
[38] Z. Ma, J.-Y. Chen, Z. Li, C. Tang, Y. M. Sua, H. Fan, Y.-P. Huang. Ultrabright quantum photon sources on chip. Phys. Rev. Lett., 125, 263602(2020).
[39] J. Zhao, C. Ma, M. Rüsing, S. Mookherjea. High quality entangled photon pair generation in periodically poled thin-film lithium niobate waveguides. Phys. Rev. Lett., 124, 163603(2020).
[40] G.-T. Xue, Y.-F. Niu, X. Liu, J.-C. Duan, W. Chen, Y. Pan, K. Jia, X. Wang, H.-Y. Liu, Y. Zhang, P. Xu, G. Zhao, X. Cai, Y.-X. Gong, X. Hu, Z. Xie, S. Zhu. Ultrabright multiplexed energy-time-entangled photon generation from lithium niobate on insulator chip. Phys. Rev. Appl., 15, 064059(2021).
[41] S. Arahira, N. Namekata, T. Kishimoto, S. Inoue. Experimental studies in generation of high-purity photon-pairs using cascaded χ(2) processes in a periodically poled LiNbO3 ridge-waveguide device. J. Opt. Soc. Am. B, 29, 434-442(2012).
[42] B. S. Elkus, K. Abdelsalam, S. Fathpour, P. Kumar, G. S. Kanter. Quantum-correlated photon-pair generation via cascaded nonlinearity in an ultra-compact lithium-niobate nano-waveguide. Opt. Express, 28, 39963-39975(2020).
[43] M. R. Billah, M. Blaicher, T. Hoose, P.-I. Dietrich, P. Marin-Palomo, N. Lindenmann, A. Nesic, A. Hofmann, U. Troppenz, M. Moehrle, S. Randel, W. Freude, C. Koos. Hybrid integration of silicon photonics circuits and InP lasers by photonic wire bonding. Optica, 5, 876-883(2018).
[44] N. Lindenmann, G. Balthasar, D. Hillerkuss, R. Schmogrow, M. Jordan, J. Leuthold, W. Freude, C. Koos. Photonic wire bonding: a novel concept for chip-scale interconnects. Opt. Express, 20, 17667-17677(2012).
[45] M. He, M. Xu, Y. Ren, J. Jian, Z. Ruan, Y. Xu, S. Gao, S. Sun, X. Wen, L. Zhou, L. Liu, C. Guo, H. Chen, S. Yu, L. Liu, X. Cai. High-performance hybrid silicon and lithium niobate Mach–Zehnder modulators for 100 Gbit s−1 and beyond. Nat. Photonics, 13, 359-364(2019).
[46] H. Okayama, M. Kawahara. Waveguide array grating wavelength demultiplexer on LiNbO3. Integrated Photonics Research, ISaB3(1995).
[47] W. Ji, Z. Gong, R. Yin, J. Li, J. Li, L. Lv, Q. Huang. Tunable arrayed waveguide grating optical filter based on lithium niobate-on-insulator and electro-optic effect. Opt. Eng., 57, 077102(2018).
[48] M. Prost, G. Liu, S. B. Yoo. A compact thin-film lithium niobate platform with arrayed waveguide gratings and MMIS. Optical Fiber Communications Conference and Exposition (OFC), 1-3(2018).
[49] H. Li, X. Cai. Flat-top CWDM using narrow straight directional couplers on LN thin film. Asia Communications and Photonics Conference (ACP) and International Conference on Information Photonics and Optical Communications (IPOC), 1-3(2020).
[50] J.-X. Zhou, R.-H. Gao, J.-T. Lin, M. Wang, W. Chu, W.-B. Li, D.-F. Yin, L. Deng, Z.-W. Fang, J.-H. Zhang, R.-B. Wu, Y. Cheng. Electro-optically switchable optical true delay lines of meter-scale lengths fabricated on lithium niobate on insulator using photolithography assisted chemo-mechanical etching. Chin. Phys. Lett., 37, 084201(2020).
[51] E. Lomonte, M. A. Wolff, F. Beutel, S. Ferrari, C. Schuck, W. H. Pernice, F. Lenzini. Single-photon detection and cryogenic reconfigurability in lithium niobate nanophotonic circuits. Nat. Commun., 12, 6847(2021).
[52] A. A. Sayem, R. Cheng, S. Wang, H. X. Tang. Lithium-niobate-on-insulator waveguide-integrated superconducting nanowire single-photon detectors. Appl. Phys. Lett., 116, 151102(2020).
[53] A. Aimone, F. Frey, R. Elschner, I. G. Lopez, G. Fiol, P. Rito, M. Gruner, A. Ulusoy, D. Kissinger, J. Fischer, C. Schubert, M. Schell. DAC-less 32-GBd PDM-256-QAM using low-power InP IQ segmented MZM. IEEE Photonics Technol. Lett., 29, 221-223(2016).
[54] C. Wang, M. Zhang, X. Chen, M. Bertrand, A. Shams-Ansari, S. Chandrasekhar, P. Winzer, M. Lončar. Integrated lithium niobate electro-optic modulators operating at CMOS-compatible voltages. Nature, 562, 101-104(2018).
[55] M. Zhang, B. Buscaino, C. Wang, A. Shams-Ansari, C. Reimer, R. Zhu, J. M. Kahn, M. Lončar. Broadband electro-optic frequency comb generation in a lithium niobate microring resonator. Nature, 568, 373-377(2019).
[56] Y. Hu, M. Yu, D. Zhu, N. Sinclair, A. Shams-Ansari, L. Shao, J. Holzgrafe, E. Puma, M. Zhang, M. Lončar. On-chip electro-optic frequency shifters and beam splitters. Nature, 599, 587-593(2021).
[57] R. H. Brown, R. Q. Twiss. Correlation between photons in two coherent beams of light. Nature, 177, 27-29(1956).
[58] R. Kumar, J. R. Ong, M. Savanier, S. Mookherjea. Controlling the spectrum of photons generated on a silicon nanophotonic chip. Nat. Commun., 5, 5489(2014).
[59] R.-B. Jin, R. Shimizu, K. Wakui, H. Benichi, M. Sasaki. Widely tunable single photon source with high purity at telecom wavelength. Opt. Express, 21, 10659-10666(2013).
[60] R.-B. Jin, J. Zhang, R. Shimizu, N. Matsuda, Y. Mitsumori, H. Kosaka, K. Edamatsu. High-visibility nonclassical interference between intrinsically pure heralded single photons and photons from a weak coherent field. Phys. Rev. A, 83, 031805(2011).
[61] X. Li, L. Yang, L. Cui, Z. Y. Ou, D. Yu. Observation of quantum interference between a single-photon state and a thermal state generated in optical fibers. Opt. Express, 16, 12505-12510(2008).
[62] A. I. Lvovsky, M. G. Raymer. Continuous-variable optical quantum-state tomography. Rev. Mod. Opt., 81, 299-332(2009).
[63] T. Pittman, B. Jacobs, J. Franson. Experimental demonstration of a quantum circuit using linear optics gates. Phys. Rev. A, 71, 032307(2005).
[64] H. Fearn, R. Loudon. Theory of two-photon interference. J. Opt. Soc. Am. B, 6, 917-927(1989).
[65] J. Rarity, P. Tapster, R. Loudon. Non-classical interference between independent sources. J. Opt. B, 7, S171-S175(2005).
[66] T. Miyazawa, K. Takemoto, Y. Nambu, S. Miki, T. Yamashita, H. Terai, M. Fujiwara, M. Sasaki, Y. Sakuma, M. Takatsu, T. Yamamoto, Y. Arakawa. Single-photon emission at 1.5 μm from an InAs/InP quantum dot with highly suppressed multi-photon emission probabilities. Appl. Phys. Lett., 109, 132106(2016).
[67] Ł. Dusanowski, M. Syperek, J. Misiewicz, A. Somers, S. Hoefling, M. Kamp, J. Reithmaier, G. Sek. Single-photon emission of InAs/InP quantum dashes at 1.55 μm and temperatures up to 80 K. Appl. Phys. Lett., 108, 163108(2016).
[68] L. Schweickert, K. D. Jöns, K. D. Zeuner, S. F. Covre da Silva, H. Huang, T. Lettner, M. Reindl, J. Zichi, R. Trotta, A. Rastelli, V. Zwiller. On-demand generation of background-free single photons from a solid-state source. Appl. Phys. Lett., 112, 093106(2018).
[69] H. Wang, Y.-M. He, T.-H. Chung, H. Hu, Y. Yu, S. Chen, X. Ding, M.-C. Chen, J. Qin, X. Yang, R.-Z. Liu, Z.-C. Duan, J.-P. Li, S. Gerhardt, K. Winkler, J. Jurkat, L.-J. Wang, N. Gregersen, Y.-H. Huo, Q. Dai, S. Yu, S. Höfling, C.-Y. Lu, J.-W. Pan. Towards optimal single-photon sources from polarized microcavities. Nat. Photonics, 13, 770-775(2019).
[70] S. K. Joshi, D. Aktas, S. Wengerowsky, M. Lončarić, S. P. Neumann, B. Liu, T. Scheidl, G. C. Lorenzo, Ž. Samec, L. Kling, A. Qiu, M. Razavi, M. Stipčević, J. G. Rarity, R. Ursin. A trusted node–free eight-user metropolitan quantum communication network. Sci. Adv., 6, eaba0959(2020).
[71] H. Zou, H. Wang. Pulse-forming-line based on-chip short pulse generator. Rev. Sci. Instrum., 86, 044706(2015).
[72] Z. Fu, H. Liu. Ultra-narrow pulse generator with precision-adjustable pulse width. Rev. Sci. Instrum., 89, 055103(2018).
[73] Z. Ou. Quantum theory of fourth-order interference. Phys. Rev. A, 37, 1607-1619(1988).
[74] F. Sun, C. Wong. Indistinguishability of independent single photons. Phys. Rev. A, 79, 013824(2009).
[75] M. Avenhaus, H. B. Coldenstrodt-Ronge, K. Laiho, W. Mauerer, I. A. Walmsley, C. Silberhorn. Photon number statistics of multimode parametric down-conversion. Phys. Rev. Lett., 101, 053601(2008).
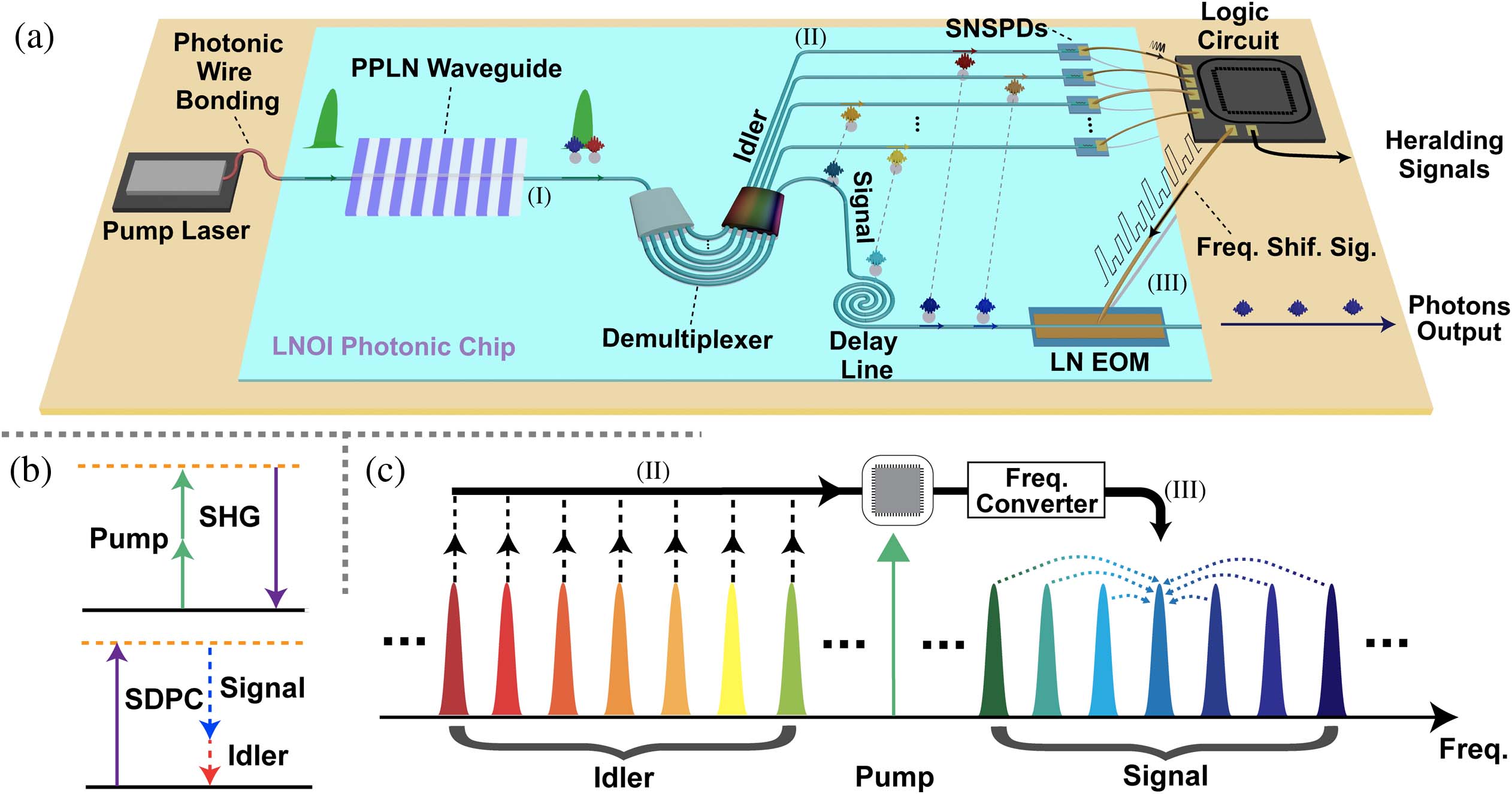
Set citation alerts for the article
Please enter your email address