
- Photonics Research
- Vol. 9, Issue 5, 722 (2021)
Abstract
1. INTRODUCTION
Optical microresonators [1], owing to their long photon storage time in small spatial volumes, enable strong enhancement of optical interactions, especially in the generation of soliton microcombs [2,3]. Specifically, the need to enhance desired nonlinear processes has motivated the development of different material platforms in improving the Q factors of such microresonators. In recent years, there has been progress made in many chip-based material platforms, such as silica [4–7], silicon nitride [8–12], lithium niobate [13,14], and others [15–22]. Among them, the chip-based silica microresonators in the forms of microtoroid, microsphere, as well as microdisk exhibit the highest Q factors [4–7]. Compared to the microtoroid and microsphere resonators, the silica microdisk resonators are more widely used for Brillouin lasers [5] and Kerr soliton microcombs [23] owing to the controllabilities of the free spectral range (FSR) and dispersion in such structures with the diameter, wedge angle, as well as thickness [23]. Also, due to the low material dispersion and ultrahigh Q factor, Kerr soliton microcombs with a repetition rate as low as 1.86 GHz have been achieved using a 35-mm-diameter microdisk resonator [24]. However, due to the fabrication limitations, such ultrahigh-Q silica microdisk resonators have only been obtained by using a chemical wet-etching technique [5,7]. In that work, extended hydrofluoric acid (HF) wet etching was performed to remove the “foot” region of the silica layer induced by the wet etching, which makes the diameter of the fabricated microresonator much smaller than the initial design [5].
In a previous work, a dry-etched silica microdisk resonator [25] with an optical Q factor of
In this work, by optimizing the fabrication process, we have obtained ultrahigh-Q silica microdisk resonators on a silicon chip by employing an inductively coupled plasma (ICP) dry-etching technique. The intrinsic optical Q factor as high as 194 million is demonstrated by fabricating a 1-mm-diameter silica microdisk resonator with a thickness of 4 μm. To the best of our knowledge, this is the first report of chip-based ultrahigh-Q silica microresonators fabricated by dry etching.
2. FABRICATION AND CHARACTERIZATION
Our standard fabrication process is similar to that shown in our previous work [25,26,28]. Compared with the HF wet etching [5], the dry-etching process usually produces larger sidewall roughness and increases the difficulty in removing photoresist, which significantly affects the Q factors of the microresonators. In this work, by improving the fabrication process such as reducing the sidewall roughness and adding a silicon layer to make the residual photoresist on top surface of the silica microdisk be easily removed, we have boosted the dry-etched silica microresonators into the ultrahigh-Q region.
So far, the optical Q factor of the chip-based silica microdisk resonator is limited by the scattering losses induced by the surface roughness of the microresonator. For the dry-etched microdisk resonators, the sidewall roughness predominantly results from two pattern-transfer steps: lithography-induced roughness and dry-etch-induced roughness. During photolithography, we optimized the hard-bake temperature and duration time of the patterned photoresist to obtain a smooth sidewall of the mask. For the dry-etching process, we employed an etching system based on neutral loop discharge (NLD) plasmas (ULVAC, NLD 570) [29]. These NLD plasmas tend to have high plasma density with a relatively low electron temperature [30], which lowers the substrate temperature and thus reduces the difficulty in subsequently removing the photoresist. This is of great importance, particularly for the long-time plasma etching in this work. During the ICP etching,
Figure 1.Typical images and atomic force microscope measurement of the ultrahigh-
Figure 2.Fabrication process flow for the dry-etched wedged silica microdisk resonators. (a) Thermally grown silica layer on a silicon chip and subsequent deposition of an a-Si layer by plasma enhanced chemical vapor deposition (PECVD). (b) Pattern definition by UV lithography. (c) ICP etching to transfer the mask pattern to the silica layer. (d) Photoresist removal. (e)
To characterize the silica microdisk resonator, a tapered optical fiber is used to excite the optical modes of the microresonator via the evanescent wave [31]. We obtain the transmission spectrum by scanning an external semiconductor laser at the 1550 nm wavelength across the resonance frequency. By adjusting the distance between the taper fiber and the resonator, the resonator can be undercoupled to avoid the thermo-optic effect [32], which makes the measured value close to the intrinsic optical Q factor. Further adjusting the distance between the taper fiber and the resonator, the critical coupling and overcoupling regimes can also be accessed. During the measurement, the optical modes are calibrated using a fiber Mach–Zehnder interferometer.
Figure 3.Comparison of intrinsic
Figure 4.Characterization of the ultrahigh-
To further confirm the measured Q factors of the microresonators, accurate measurement using the ringing method [35,36] was also performed to minimize the influence of thermal effects and laser frequency noise. The laser excites the optical mode, which results in the interference between the delayed light from the resonator and the direct transmitted pump laser. Figure 4(b) shows the ringing curve of the optical mode in Fig. 4(a). The theoretical fit shows an intrinsic Q factor of 196.2 million, which matches quite well with the Lorentzian fit in Fig. 4(a). Particular attention should be paid to the fact that the Q factor is even higher than that for a chemically etched analogue with the same diameter and thickness [5].
Figure 5.Sidebands’ power versus input pump power. The measured threshold is 1.63 mW for the silica microdisk. The inset shows the optical spectrum with the pump power of 1.66 mW.
To evaluate the surface roughness of the fabricated microdisk resonators, we have performed atomic force microscope (AFM) measurement. As shown in Figs. 1(c) and 1(d), the root-mean-squared (RMS) roughness is 0.72 nm for the top surface and 0.67 nm for the sidewall. The relatively larger roughness of the top surface may be attributed to the residual photoresist or silicon. The roughness of the sidewall is similar to the measured value of the wet-etched silica microdisk resonator [5]. Also, the autocorrelation lengths for the top surface and the sidewall are around 20 nm and 30 nm, respectively, which are much smaller than that obtained from wet etching [5]. In the future, the optical Q factor can be further increased by optimizing the fabrication process such as the removal of the photoresist on the top surface of the microresonator.
3. CONCLUSION
In conclusion, ultrahigh-Q (
Acknowledgment
Acknowledgment. The authors thank Jiayu Zhang, Hongyu Yang, and Kuo Yang for assistance with AFM measurement.
References
[1] K. J. Vahala. Optical microcavities. Nature, 424, 839-846(2003).
[2] T. J. Kippenberg, A. L. Gaeta, M. Lipson, M. L. Gorodetsky. Dissipative Kerr solitons in optical microresonators. Science, 361, 8083(2018).
[3] P. Del’Haye, A. Schliesser, O. Arcizet, T. Wilken, R. Holzwarth, T. Kippenberg. Optical frequency comb generation from a monolithic microresonator. Nature, 450, 1214-1217(2007).
[4] D. Armani, T. Kippenberg, S. Spillane, K. Vahala. Ultra-high-
[5] H. Lee, T. Chen, J. Li, K. Y. Yang, S. Jeon, O. Painter, K. J. Vahala. Chemically etched ultrahigh-
[6] J.-B. Jager, V. Calvo, E. Delamadeleine, E. Hadji, P. Noé, T. Ricart, D. Bucci, A. Morand. High-
[7] L. Wu, H. Wang, Q. Yang, Q. Ji, B. Shen, C. Bao, M. Gao, K. Vahala. Greater than one billion
[8] Y. Xuan, Y. Liu, L. T. Varghese, A. J. Metcalf, X. Xue, P.-H. Wang, K. Han, J. A. Jaramillo-Villegas, A. Al Noman, C. Wang, S. Kim, M. Teng, Y. J. Lee, B. Niu, L. Fan, J. Wang, D. E. Leaird, A. M. Weiner, M. Qi. High-
[9] D. T. Spencer, J. F. Bauters, M. J. Heck, J. E. Bowers. Integrated waveguide coupled Si3N4 resonators in the ultrahigh-
[10] X. Ji, F. A. Barbosa, S. P. Roberts, A. Dutt, J. Cardenas, Y. Okawachi, A. Bryant, A. L. Gaeta, M. Lipson. Ultra-low-loss on-chip resonators with sub-milliwatt parametric oscillation threshold. Optica, 4, 619-624(2017).
[11] M. H. Pfeiffer, J. Liu, A. S. Raja, T. Morais, B. Ghadiani, T. J. Kippenberg. Ultra-smooth silicon nitride waveguides based on the Damascene reflow process: fabrication and loss origins. Optica, 5, 884-892(2018).
[12] M. H. Pfeiffer, A. Kordts, V. Brasch, M. Zervas, M. Geiselmann, J. D. Jost, T. J. Kippenberg. Photonic Damascene process for integrated high-
[13] M. Zhang, C. Wang, R. Cheng, A. Shams-Ansari, M. Lončar. Monolithic ultra-high-
[14] A. Boes, B. Corcoran, L. Chang, J. Bowers, A. Mitchell. Status and potential of lithium niobate on insulator (LNOI) for photonic integrated circuits. Laser Photon. Rev., 12, 1700256(2018).
[15] B. Hausmann, I. Bulu, V. Venkataraman, P. Deotare, M. Lončar. Diamond nonlinear photonics. Nat. Photonics, 8, 369-374(2014).
[16] M. Pu, L. Ottaviano, E. Semenova, K. Yvind. Efficient frequency comb generation in AlGaAs-on-insulator. Optica, 3, 823-826(2016).
[17] Z. Gong, A. Bruch, M. Shen, X. Guo, H. Jung, L. Fan, X. Liu, L. Zhang, J. Wang, J. Li, J. Yan, H. X. Tang. High-fidelity cavity soliton generation in crystalline AlN micro-ring resonators. Opt. Lett., 43, 4366-4369(2018).
[18] D. J. Wilson, K. Schneider, S. Hönl, M. Anderson, Y. Baumgartner, L. Czornomaz, T. J. Kippenberg, P. Seidler. Integrated gallium phosphide nonlinear photonics. Nat. Photonics, 14, 57-62(2020).
[19] X. Lu, J. Y. Lee, S. Rogers, Q. Lin. Optical Kerr nonlinearity in a high-
[20] L. Chang, W. Xie, H. Shu, Q.-F. Yang, B. Shen, A. Boes, J. D. Peters, W. Jin, C. Xiang, S. Liu, G. Moille, S.-P. Yu, X. Wang, K. Srinivasan, S. B. Papp, K. Vahala, J. E. Bowers. Ultra-efficient frequency comb generation in AlGaAs-on-insulator microresonators. Nat. Commun., 11, 1331(2020).
[21] A. Biberman, M. J. Shaw, E. Timurdogan, J. B. Wright, M. R. Watts. Ultralow-loss silicon ring resonators. Opt. Lett., 37, 4236-4238(2012).
[22] S. A. Miller, M. Yu, X. Ji, A. G. Griffith, J. Cardenas, A. L. Gaeta, M. Lipson. Low-loss silicon platform for broadband mid-infrared photonics. Optica, 4, 707-712(2017).
[23] X. Yi, Q.-F. Yang, K. Y. Yang, M.-G. Suh, K. Vahala. Soliton frequency comb at microwave rates in a high-
[24] M.-G. Suh, K. Vahala. Gigahertz-repetition-rate soliton microcombs. Optica, 5, 65-66(2018).
[25] G. Li, P. Liu, X. Jiang, C. Yang, J. Ma, H. Wu, M. Xiao. High-
[26] J. Ma, L. Xiao, J. Gu, H. Li, X. Cheng, G. He, X. Jiang, M. Xiao. Visible Kerr comb generation in a high-
[27] C. Pyrlik, J. Schlegel, F. Böhm, A. Thies, O. Krüger, O. Benson, A. Wicht, G. Tränkle. Integrated thermal silica micro-resonator waveguide system with ultra-low fluorescence. IEEE Photon. Technol. Lett., 31, 479-482(2019).
[28] X. Jiang, Q. Lin, J. Rosenberg, K. Vahala, O. Painter. High-
[29] T. Uchida. Application of radio-frequency discharged plasma produced in closed magnetic neutral line for plasma processing. Jpn. J. Appl. Phys., 33, L43-L44(1994).
[30] T. Uchida, S. Hamaguchi. Magnetic neutral loop discharge (NLD) plasmas for surface processing. J. Phys. D, 41, 083001(2008).
[31] M. Cai, O. Painter, K. J. Vahala. Observation of critical coupling in a fiber taper to a silica-microsphere whispering-gallery mode system. Phys. Rev. Lett., 85, 74-77(2000).
[32] T. Carmon, L. Yang, K. J. Vahala. Dynamical thermal behavior and thermal self-stability of microcavities. Opt. Express, 12, 4742-4750(2004).
[33] T. Kippenberg, S. Spillane, K. Vahala. Modal coupling in traveling-wave resonators. Opt. Lett., 27, 1669-1671(2002).
[34] M. L. Gorodetsky, A. D. Pryamikov, V. S. Ilchenko. Rayleigh scattering in high-
[35] A. A. Savchenkov, A. B. Matsko, V. S. Ilchenko, L. Maleki. Optical resonators with ten million finesse. Opt. Express, 15, 6768-6773(2007).
[36] C. Dong, C. Zou, J. Cui, Y. Yang, Z. Han, G. Guo. Ringing phenomenon in silica microspheres. Chin. Opt. Lett., 7, 299-301(2009).
[37] K. Y. Yang, K. Beha, D. C. Cole, X. Yi, P. Del’Haye, H. Lee, J. Li, D. Y. Oh, S. A. Diddams, S. B. Papp, K. J. Vahala. Broadband dispersion-engineered microresonator on a chip. Nat. Photonics, 10, 316-320(2016).
[38] H. Lee, T. Chen, J. Li, O. Painter, K. J. Vahala. Ultra-low-loss optical delay line on a silicon chip. Nat. Commun., 3, 867(2012).
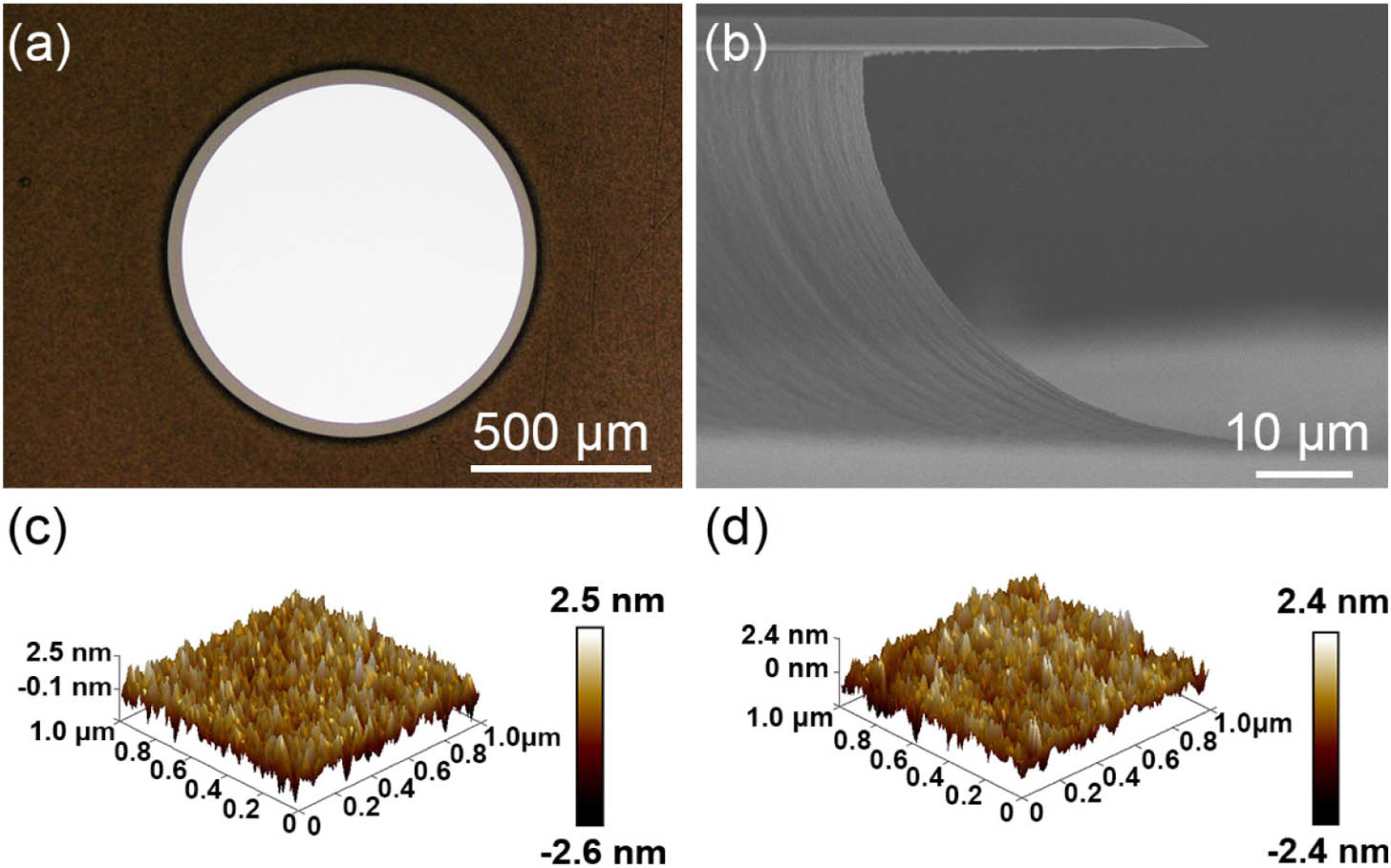
Set citation alerts for the article
Please enter your email address