
- Journal of Semiconductors
- Vol. 41, Issue 7, 071901 (2020)
Abstract
1. Introduction
Silicon (Si) based technologies are the basis of complementary metal oxide semiconductor (CMOS) field effect transistor (FET) devices and they are the main driving force for the rapid growth of information technology[
Contact resistance is critical for achieving high performance and low power consumption in Si-based CMOS FET or advanced 2D SCs based integrated circuits. Contacts are the communication links between these 2D SCs and external circuitry. The properties of the interface between metal–2D SCs govern the performance of these devices, including drain current[
In this review, the state-of-the-art studies of contact engineering of 2D SCs are summarized. We first introduce the background of 2D materials. Next, we discuss the contact geometry and the nature of metal-2D SCs contact and describe the Fermi level pinning and Schottky barrier of metal-2D SCs briefly. Then, conventional metal contacts and metallic 2D materials contacts are discussed, where the advantages and disadvantages of each method are presented and illustrated by some examples. Especially, using metallic 2D materials as contacts is discussed in details in this review. Finally, the challenges and under-explored aspects of the contact engineering of 2D SCs are proposed.
2. Development of 2D SCs
Layered materials, which have a strong covalent bonding within the layer and weak vdW interaction between the layers, have been widely studied thanks to their fascinating properties[
Figure 1.(Color online) Atomic structure of representative 2D semiconductor materials and phase engineered contacts. (a, b) Top and side view of 2H stacked structure of 2D semiconductor. (c) Device model of 2D SCs based device. (d–f) Different types of metal–semiconductor junction with contact interface.
3. Contact geometry and Schottky barrier of a metal-2D SC contact
3.1. Contact geometry
The field effect transistor (FET) is the most fundamental and common unit for electronic devices used in fundamental research and industry, as shown in Fig. 1(c). Heavily doped Si is used as the conductive substrate, where the back gated voltage is applied. The thermally oxidized SiO2 on Si substrate with thickness in the range of 90–300 nm is a dielectric layer and the 2D SCs can either be mechanically exfoliated or CVD grown onto the substrate. As the 3D top contact, the surface of bulk (3D) SCs tends to form covalent bonds with metals, shown in Fig. 1(d). However, for the metal-2D SC contact, it is difficult to form covalent bonds on the surface of 2D SCs. There will be a van der Waals gap between the interface of the metal (such as Au) and 2D SCs[
Although 3D top contact is commonly used between metal electrode and 2D SCs (Fig. 2(a)), considering the disadvantages of such contact, a 3D edge contact is proposed to overcome the Fermi level pinning effect at the interface (Fig. 2(b)). Both experiments and DFT calculation have shown the numerous advantageous of 3D edge contacts[
Figure 2.(Color online) (a, b) 3D top contact and 3D edge contact configuration of metal-2D SCs contact. (c, d) 2D top contact and 2D edge contact of metallic 2D-SCs contact geometries.
3.2. Schottky barrier of a metal-2D SC contact
In traditional Si-based CMOS integrated circuits, ohmic contacts are realized by heavy ion diffusion or implantation in body Si substrate. A simplified schematic diagram of an n-type MOS (NMOS) FET is shown in Fig. 3. The NMOS FET is fabricated on the p-type Si substrate, where the thickness of body Si is less than 1 mm generally. The Leff and tox are the effective channel length and thickness of oxide, and the typical value of which are about 0.15 μm and 50 Å, respectively[
Figure 3.(Color online) Schematic diagram of a conventional Si-based NMOS FET.
where J and V is current density and external bias voltage, respectively. For metal-SCs contact with low doping concentration of SCs, the thermionic emission current dominates. The characteristic of J–V can be represented as[
where Js, A*, T, k and
From Eq. (4), it can be found that Rc is proportion to
where ND, mn,
From Eq. (7), a small Rc can be realized by high doping concentration. As aforementioned, in conventional Si-based CMOS circuits, ohmic contacts are realized by heavy doping. However, when Si is substituted by 2D SCs, such controllable and sustainable doping strategies are not applicable to the 2D SCs based FET because of their fragility. Most contacts between metal and 2D SCs are Schottky contacts, which will increase contact resistance and limit the carrier injection. Therefore, reducing the ФSB of metal and 2D SCs contact is an effective way to improve carrier injection efficiency.
Fig. 4(a) shows a schematic diagram of several metal-MoS2 band alignments along with work function of metal (ФM)[
Figure 4.(Color online) (a) Schematic diagram of various metal–MoS2 band alignments along with work function of metal (
where ФSB-n and ФSB-p are Schottky barrier heights for electrons and holes, respectively. Eg are bandgaps of the 2D SCs. Ideally, all of the parameters are followed by a Schottky–Mott rule and ФSB is linearly dependent on the ФM with a slope of unity. However, in fact, an ideal metal–2D SCs contact rarely forms and the ФSB is often incorrect estimated by Schottky–Mott mode, which was first noted by Bardeen in 1947[
where ФIS and S are the interface state energy of SC and Schottky pinning factor, respectively. If S = 0, then ФSB is independent of metal ФM, which indicates the strong pinning effect at the metal–SC interface. If S = 1, then ФSB is determined by Eqs. (1) and (2), and the Schottky–Mott limit is achieved. In the case of MoS2 and Ti contact, the theoretical value of ФSB is about 0.1 eV according to Eq. (8). The actual ФSB from experiment is about 50 meV[
4. Conventional metal contacts and 2D metallic material contacts
In this part, we first discuss the strategies of conventional metal contacts (3D top contact and 3D edge contact) to decrease the contact resistance and improve carrier injection efficiency, including selecting matched contact metal, designing contact geometry, introducing special metal interlayer and metal transfer approaches. Next, strategies to introduce 2D metallic materials contacts (2D top contact and 2D edge contact) are discussed in detail, such as CVD growth of 2D metallic materials as contact, phase engineering of 2D SCs to metallic phase as contact. Finally, we discuss an intercalation strategy to induce metallic state.
4.1. Conventional metal contacts
4.1.1. Select matched contact metal
Due to the lack of controllable and sustainable doping strategies for 2D SCs FET, the contact quality and resistance are determined by the contact metal. Therefore, a proper understanding and choice of source/drain contacts metals is essential to improve the performance of 2D SCs-based FETs. Das et al. presented a thorough experimental study of contacts to MoS2 by using different ФM metals such as Sc (ФM = 3.5 eV), Ti (ФM = 4.3 eV), Ni (ФM = 5.3 eV) and Pt (ФM = 5.6 eV)[
Figure 5.(Color online) Metal contact investigation of MoS2 device. (a) Schematic diagram of back gated MoS2 transistor. (b, c) SEM and AFM images of device with MoS2 thickness of 3 nm. (d) The expected position of the metal Fermi level across the band of MoS2, where the
Fig. 5(f) shows the temperature-dependent transfer curves with Ni contact, which was used to create the Arrhenius plot in Fig. 5(g). The actual ФSB can be revealed by the Arrhenius plot if the flat band voltage VFB is identified, and the VFB has been marked in Fig. 5(g). Note that the contributions of thermionic emission and thermally assisted tunneling are identified at different Vgs in Fig. 5(d). Based on the conventional thermionic emission theory, the slope of the curves in high-temperature region is presented. The ФSB is extracted by equation[
where Ids, A, kB, q, T and Vds are the current through the device, Richardson’s constant, Boltzmann constant, electronic charge, temperature and source to drain bias, respectively. From Fig. 5(h), the extracted ФSB of Ni-contacted MoS2 is 150 meV, much lower than that calculated from Schottky–Mott rule. Fig. 5(i) shows the extracted ФSB from different metals with MoS2 as a function of their corresponding ФM. The ФSB is 30, 50, 150, and 230 meV for Sc, Ti, Ni, and Pt, respectively. The slope extracted by dФSB/dФM (interface parameter S) is about 0.1, which indicates strong Fermi level pinning effects at the metal–MoS2 interface and the Fermi levels of metal are pinned close to the conduction band in the bandgap of MoS2. It also explains why all of the metal contacts present n-type characteristics, even when using the large ФM metal. The carrier mobility of MoS2 with thickness of 10 nm is 184, 125, 36, and 21 cm2/(V·s) for Sc, Ti, Ni and Pt contact. However, the mobility is less than 20 cm2/(V·s) for Sc contact when the thickness of MoS2 is reduced to 3 nm. The results indicate that the performance of monolayer or few layer MoS2 FET can be further improved more effectively, except for the selection of the matched contact metal.
4.1.2. Introducing a special interlayer metal
Although the performance of 2D SCs FET can be improved by proper contact metal, the Fermi level pinning effect at the interface still limits the development of 2D SCs FETs. Creating van-der-Waals-type bonding between three-dimensional metal and 2D SCs is an ingenious method to improve the performance of 2D SCs FET. In a van-der-Waals-type contact, the contact resistance and ФSB can be decreased substantially. Furthermore, the Fermi level pinning effect at the metal–2D SCs interface can effectively be avoided[
Fig. 6(a) shows a schematic of the In/Au contacted MoS2 FET device. Cross-sectional annular dark field (ADF) scanning transmission electron microscopy (STEM) was used to verify the interface between In/Au–MoS2. Fig. 6(b) shows ADF and bright-field STEM images. The atomic resolution cross-sectional image of In/Au and MoS2 indicates that monolayer MoS2 is atomically sharp with no detectable evidence of reaction with In/Au. In addition, there is no observed damage on MoS2, indicating that In is gently deposited on the surface of MoS2. These results demonstrate the presence of a high-quality contact interface through the formation of van-der-Waals-type bonding of MoS2 and In layer. To investigate the performance of In/Au contacted MoS2 FET, contact resistance and FET properties are measured. Figs. 6(c) and 6(d) show the measured contact resistance of CVD growth monolayer (0.7 nm) and mechanically exfoliated few-layer (8 nm) MoS2 FETs using transmission line method (TLM). The contact resistance is 3.3 ± 0.3 kΩ·μm (at n = 5.0 × 1012 cm−2) and 800 ± 200 Ω·μm (at n = 3.1 × 1012 cm−2), respectively. Figs. 6(e) and 6(f) compare the contact resistance from the literature, indicating that the contact resistances achieved in this work are the lowest among all carrier concentrations. Figs. 6(g) and 6(h) present the room temperature and temperature-dependent transfer characteristics of monolayer and few-layer MoS2 FETs, respectively. A mobility of about 170 cm2/(V·s) can be achieved by using In/Au electrodes with monolayer MoS2 FET. Fig. 6(i) shows the output curves of monolayer MoS2 FET, where the linear output characteristics indicate the absence of a contact barrier and ohmic contact. The ФSB extracted from few-layer MoS2 in Fig. 6(j) is about 110 meV, which is consistent with the difference between work function of the metal and the conduction-band energy level of MoS2. Furthermore, the achieved ideal contact demonstrates that the Fermi level pinning effect is absent at this interface. In conclusion, this work provides a simple method to make ultraclean van der Waals contacts with standard industrial technology.
Figure 6.(Color online) In contacted MoS2 transistors. (a) Device mode of MoS2 based back-gated device. The electrodes consist of 10 nm In layer capped with 100 nm Au. (b) Cross-sectional annular dark field (ADF) scanning transmission electron microscopy (STEM) of interface of In-MoS2. (c, d) Extracted contact resistance using transmission line method for MoS2 with thickness of 0.7 and 8.1 nm, respectively. (e, f) Comparison of contact resistance
4.1.3. Transfer metal electrode
Another approach to improve the performance of 2D SCs FET is to transfer the electrode to the surface of 2D SCs, which has recently been reported by Duan et al.[
Figure 7.(Color online) Transferred metal electrode contacted MoS2 FET. (a–c) Cross-sectional atomic mode and optical images of MoS2 device with transferred Au and the transferred Au mechanically released. (d–f) Cross-sectional schematics and optical images of MoS2 device with traditional electron-beam-deposited Au and the deposited Au mechanically released. (g, h) Cross-sectional schematics and TEM images of transferred and evaporated Au. (i, j) Transfer curves of MoS2 devices with different transferred and deposited metals. (k) Experimentally extracted
Fig. 7(i) shows the transfer characteristics of MoS2 with electron-beam-deposited metal electrode. All of the devices present n-type behavior irrespective of metals with high or low ФM, which is consistent with previous studies[
4.1.4. Metal edge contact
Considering the aforementioned limits of a 3D top contact, 3D edge contact has proven to be highly advantageous when compared with a 3D top contact in terms of carrier injection efficiency[
Figure 8.(Color online) Edge contact investigation of MoS2 device. (a) Schematic diagram of hBN encapsulated MoS2 device with graphene edge contact. (b–d) Temperature dependent output curves, contact resistance and temperature dependent Hall mobility, respectively[
Note that the edge contact in Fig. 8(a) is bridged by graphene and is not directly edge contacted with 2D SCs. Chai et al. fabricated the pure edge contacted MoS2 device through pre-deposition Al2O3 by ALD or encapsulated h-BN on MoS2[
4.2. Metallic 2D material contacts
Apart from conventional metal contact, integrating 2D metallic materials on SCs to form van der Waals contact is also an attractive way to eliminate Fermi level pinning effect and decrease the contact resistance. Furthermore, 2D metallic-SCs heterostructures have wide potential in future applications, such as high-performance transistors[
Besides CVD growth method, phase engineering and intercalation can also introduce 2D metal–SCs in-plane heterostructure. The metallic state can be realized by point defects through laser or Ar plasma treatment, or introducing interlayer metal atoms through intercalation approaches, which is similar to the heavy doping technology in Si-based CMOS circuits. Therefore, this type of 2D SCs FET can be regarded as a simplified conventional Si-based NMOS FET. The difference is that doping technology in Si-based CMOS circuits is controllable and sustainable, while doping strategies for 2D SCs FET can only be applied to certain 2D SCs.
4.2.1. CVD growth 2D metallic materials as contact
The CVD method can produce many kinds of 2D metallic-SCs heterostructures with high yield, including the stacking orientation controlled vertical and seamless lateral 2D metallic-SCs heterostructures[
Recently, Lee et al. and Duan et al. achieved heteroepitaxial stacking of van der Waals monolayer 1T metallic-2H SC WSe2 heterostructures, in which a distinctively low contact barrier was achieved across a clean epitaxial van der Waals gap[
Figure 9.(Color online) Contact engineering of vertical 2D metallic-2H WSe2 heterostructure. (a) Sequential growth scheme of vertical WSe2 and WT2 heterostructure. The inset is the optical images of WSe2 and WTe2. (b) Device mode of WSe2 with and without contact. (c, d) Output curves and temperature-dependent conductivity of WSe2 device with WTe2 and Ti contact. (e) Built-in potential energy versus back-gated voltage for Ti and WTe2 contacted WSe2 devices. (f)
Lateral 2D metallic-SCs heterostructures are also utilized to fabricate device with 2D metallic material contact. Figs. 10(a) and 10(e) show a schematic model of lateral graphene–MoS2 heterostructures[
Figure 10.(Color online) Contact engineering of lateral graphene–MoS2 and VS2–MoS2 heterostructures. (a) Schematic diagram of MoS2–graphene lateral heterostructure. (b, c) Transfer and output curves of graphene contacted MoS2 device. (d) Statistics of obtained mobility from graphene contacted MoS2 device[
4.2.2. Phase engineering 2D semiconductor into metallic phase
Compared to CVD grown 2D metallic-SCs heterostructures, the phase engineering method is more specific. On the one hand, it can only be applied to 2D SCs that have two or more stable or metastable phases; while on the other hand, the phase transition region can be patterned selectively. For example, the stable phase on MoS2 is trigonal prismatic (H) coordination and a semiconductor while the MoS2 with octahedral (T) coordination is a metastable phase with metallic nature[
Electron donation induced phase transition—such as n-butyl lithium treatment[
Figure 11.(Color online) Electron donation, laser or Ar plasma induced metallic phase as contact. (a) Electrostatic force microscopy phase image of 1T–2H–1T lateral MoS2 heterostructure. (b–d) Resistance, output and transfer curves of 2H phase MoS2 with metallic 1T phase MoS2 contact. The inset is the device model of MoS2 device with Au and 1T metallic MoS2 contact[
4.2.3. Intercalation induced metallic state
Intercalation is a convenient and promising method to tune the properties of van der Waals materials[
Figure 12.(Color online) Intercalation induced phase transition of SnS2. (a) and (b) Atomic structures of pristine SnS2, Cu and Co intercalated SnS2. (c–f) Optical images of pure SnS2, Cu, Co and Cu–Co intercalated SnS2. (g) Temperature-dependent resistance of SnS2, Cu–SnS2, Co–SnS2 and few layer graphene, where the Cu–SnS2 presents semiconductor behavior while the Co–SnS2 behaves as a metal like graphene. (h) Transfer curves of Cu–SnS2 with p-type characteristic. (i)
5. Summary and outlook
In summary, in this review we have discussed the fundamental aspects related to contacts of 2D materials devices, including contact geometry and Schottky barrier. We also presented the progress of contact engineering strategies to improve the contact quality. In the conventional metal contact part, we show that the performance of 2D SCs FET can be improved by the right choice of top contact metal. However, the Fermi level pinning effect at the interface cannot be avoided, resulting in Schottky contact between top metal and 2D SCs. Fortunately, an ohmic contact can be realized and the Fermi level pinning effect can be eliminated by van-der-Waals-type bonding or gap through introduction of an In interlayer or metal electrode transfer method. A 3D edge contact was also emphasized and developed because it is advantageous over 3D top contact in terms of carrier injection efficiency, even if the pure edge contact technology is in the initial stage. However, the fabrication process of these 3D top contact or edge contact method is still complicated and faces the difficulties of scalable production.
Compared to traditional metal contact, a van-der-Waals-type metallic contact can easily be achieved by 2D metallic materials grown through CVD method. The performance of 2D SCs FET has improved substantially owing to the elimination of Fermi level pinning effect and reduction of ФSB and contact resistance. But the properties of 2D SCs may be degraded after the growth of 2D metallic materials because the defects and impurities may be introduced in 2D SC’s lattice and surface at high temperature. This disadvantage can be avoided by phase engineering or intercalation methods. The phase engineering method is an effective way to achieve ohmic contact for 2D SCs FET. The problem of this method is that the induced T phase is unstable and may return to the H phase as time goes on or at temperatures above 100 °C. In contrast, transition metal intercalation induced metallic state is very stable, which makes it a promising post-growth treatment method to fabricate spatially controlled atomically seamless in-plane 2D metallic-SCs heterostructure. The limitation of the intercalation method is that it cannot be applied to all the 2D materials and the interfaces of the resulted heterostructures have not been well characterized. If the intercalation method can be further developed and applied to those 2D SCs with strong interlayer van der Waals coupling, for example double layer MoS2 or WS2, then it will be a tremendous advance and will facilitate the application of this method to 2D SCs based transistors.
Although great progress has been achieved in the field of contact engineering for 2D SCs, there are still some challenges to solve. First, the origin of Fermi level pinning effect is still in debate. Some possible mechanisms, such as charge redistribution induced interface dipoles at interface of metal and 2D SCs or contact metal and chalcogen interaction caused orbital overlap are believed to be responsible for the Fermi level pinning effect[
Second, phase engineering or intercalation method induced 2H–1T in-plane heterostructure has previously been reported but, up to now, the barrier at 2H–1T boundary has not been systematically studied. It is necessary to propose a method to predict or calculate the boundary barrier, which will guide us to fabricate high quality heterostructure with ignored tunneling width for carrier injection across the boundary.
Third, it is well known that 2D materials with excellent optical and electrical properties are promising alternatives of Si in future applications in CMOS integrated circuits, and the device dimensions can be further scaled down owing to the atomically thin nature. However, up to now, all the contact engineering strategies reported are limited to a few 2D materials. There are no universal and scalable strategies that can be applied to a highly scaled device in a manufactural way. Before the practical application of contact engineering strategies in modern circuits, wafer scale single crystal with thickness controlled 2D SCs must be made. CVD is one of the most promising methods to get wafer-scale 2D materials, but well crystallinity, repeatability and controllability are still hard to obtain. In addition, an accurate and suitable method should be exploited to precisely control the nucleation position of heterostructures. Very recently, Duan et al. provided a general scalable synthesis method to precisely control the nucleation position and growth process of 2D metallic-SCs heterostructures[
Figure 13.(Color online) General scalable synthesis of precisely controlled nucleation position and growth process of 2D metallic-SCs heterostructures. (a) Schematic process of selectively patterned periodic defect arrays of 2D SCs (MoS2, WSe2 and WS2) to grow 2D metallic materials (VSe2, VS2, CoTe2, NiTe2 and NbTe2). (b) Schematic diagram of VSe2 growth on patterned sites of WSe2. (c–e) Optical images of periodical arrays of 2D metallic materials on WSe2. (f) Optical image of VSe2 contacted WSe2 FET periodical arrays. (g–j) Electrical characterizations of the VSe2 contacted WSe2 FET[
Acknowledgements
This work was supported by the National Key R&D Program of China (Grant No. 2018YFA0306900) and the Natural Science Foundation of China (Grant No. 51872012).
References
[1] R Schaller. Moore's law: past, present and future. IEEE Spectrum, 34, 52(1997).
[2] D J Frank, R H Dennard, E Nowak et al. Device scaling limits of Si MOSFETs and their application dependencies. Proc IEEE, 89, 259(2001).
[3] D Sarkar, X J Xie, W Liu et al. A subthermionic tunnel field-effect transistor with an atomically thin channel. Nature, 526, 91(2015).
[4] A J Arnold, A Razavieh, J R Nas et al. Mimicking neurotransmitter release in chemical synapses via hysteresis engineering in MoS2 transistors. ACS Nano, 11, 3110(2017).
[5] Y J Gong, G Shi, Z H Zhang et al. Direct chemical conversion of graphene to boronand nitrogen-and carbon-containing atomic layers. Nat Common, 5, 3193(2014).
[6] Y L Xie, B Lian, B Jäck et al. Spectroscopic signatures of many-body correlations in magic-angle twisted bilayer graphene. Nature, 572, 101(2019).
[7] L F Li, W Liu, A Y Gao et al. Plasmon excited ultrahot carriers and negative differential photoresponse in a vertical graphene van der Waals heterostructure. Nano Lett, 19, 3295(2019).
[8] K S Novoselov, A K Geim, S V Morozov et al. Two-dimensional gas of massless Dirac fermions in graphene. Nature, 438, 197(2005).
[9] Y B Zhang, Y W Tan, H L Stormer et al. Experimental observation of the quantum Hall effect and Berry's phase in graphene. Nature, 438, 201(2005).
[10] C Lee, X D Wei, J W Kysar et al. Measurement of the elastic properties and intrinsic strength of monolayer graphene. Science, 321, 385(2008).
[11] Y P Liu, I Yudhistira, M Yang et al. Phonon-mediated colossal magnetoresistance in graphene/black phosphorus heterostructures. Nano Lett, 18, 3377(2018).
[12] S Y Huang, G W Zhang, F R Fan et al. Strain-tunable van der Waals interactions in few-layer black phosphorus. Nat Common, 10, 2447(2019).
[13] K Chaudhary, M Tamagnone, M Rezaee et al. Engineering phonon polaritons in van der Waals heterostructures to enhance in-plane optical anisotropy. Sci Adv, 5, eaau7171(2019).
[14] J M Kim, S S Baik, S H Ryu et al. Observation of tunable band gap and anisotropic Dirac semimetal state in black phosphorus. Science, 349, 723(2015).
[15] R M Peng, K Khaliji, N Youngblood et al. Midinfrared electro-optic modulation in few-layer black phosphorus. Nano Lett, 17, 6315(2017).
[16] Z Liu, Y G Gong, W Zhou et al. Ultrathin high-temperature oxidation-resistant coatings of hexagonal boron nitride. Nat Commun, 4, 2541(2013).
[17] E X Wu, Y Xie, J Zhang et al. Dynamically controllable polarity modulation of MoTe2 field-effect transistors through ultraviolet light and electrostatic activation. Sci Adv, 5, eaav3430(2019).
[18] H J Park, R Y J Tay, X Wang et al. Double-spiral hexagonal boron nitride and shear strained coalescence boundary. Nano Lett, 19, 4229(2019).
[19] L Song, L J Ci, H Lu et al. Large scale growth and characterization of atomic hexagonal boron nitride layers. Nano Lett, 10, 3209(2010).
[20] L J Ci, L Song, C J Jin et al. Atomic layers of hybridized boron nitride and graphene domains. Nat Mater, 9, 430(2010).
[21] Y G Gong, J H Lin, X L Wang et al. Vertical and in-plane heterostructures from WS2/MoS2 monolayers. Nat Mater, 13, 1135(2014).
[22] Y G Gong, Z Liu, A R Lupini et al. Band gap engineering and layer-by-layer mapping of selenium doped molybdenum disulfide. Nano Lett, 14, 442(2014).
[23] Y Ma, P M Ajayan, Y J Gong et al. Recent advances in synthesis and applications of 2D junctions. Small, 14, 1801606(2018).
[24] Y C Lin, S S Li, H P Komsa et al. Revealing the atomic defects of WS2 governing its distinct optical emissions. Adv Funct Mater, 28, 1704210(2017).
[25] L F Sun, W S Leong, S Z Yang et al. Concurrent synthesis of high-performance monolayer transition metal disulfdes. Adv Funct Mater, 27, 1605896(2017).
[26] Y Zhou, H J Jang, J M Woods et al. Direct synthesis of large-scale WTe2 thin films with low thermal conductivity. Adv Funct Mater, 27, 1605928(2017).
[27] Y D Zhao, J S Qiao, P Yu et al. Extraordinarily strong interlayer interaction in 2D layered PtS2. Adv Mater, 28, 2399(2017).
[28] J X Wu, Y J Liu, Z J Tan et al. Chemical patterning of high-mobility semiconducting 2D Bi2O2Se crystals for integrated optoelectronic devices. Adv Mater, 29, 1704060(2017).
[29] L Li, Y C Guo, Y P Sun et al. A general method for the chemical synthesis of large-scale, seamless transition metal dichalcogenide electronics. Adv Mater, 30, 1706215(2018).
[30] Y H Huan, J P Shi, X L Zou et al. Vertical 1T-TaS2 synthesis on nanoporous gold for high-performance electrocatalytic applications. Adv Mater, 30, 1705916(2018).
[31] T Zhang, L Fu. Controllable chemical vapor deposition growth of two-dimensional heterostructures. Chem, 4, 671(2018).
[32] R J Xu, H Jang, M H Lee et al. Vertical MoS2 double-layer memristor with electrochemical metallization as an atomic-scale synapse with switching thresholds approaching 100 mV. Nano Lett, 19, 2411(2019).
[33] Y B Zhu, Y J Li, R A Arefe et al. Monolayer molybdenum disulfide transistors with single-atomthick gates. Nano Lett, 18, 3807(2018).
[34] S Kim, Z P Yao, J M Lim et al. Atomic-scale observation of electrochemically reversible phase transformations in SnSe2 single crystals. Adv Mater, 30, 1804925(2018).
[35] C S Liu, X Yan, X F Song et al. A semi-floating gate memory based on van der Waals heterostructures for quasi-non-volatile applications. Nat Nano, 13, 404(2018).
[36] A Y Gao, J W Lai, Y J Wang et al. Observation of ballistic avalanche phenomena in nanoscale vertical InSe/BP heterostructures. Nat Nano, 14, 217(2019).
[37] S Das, J A Robinson, M Dubey et al. Beyond graphene: progress in novel two dimensional materials and van der Waals solids. Annu Rev Mater Res, 45, 1(2015).
[38] V K Sangwan, M E Beck, A Henning et al. Self-aligned van der Waals heterojunction diodes and transistors. Nano Lett, 18, 1421(2018).
[39] D Lembke, A Kis. Breakdown of high-performance monolayer MoS2 transistors. ACS Nano, 6, 10070(2012).
[40] S Manzeli, D Ovchinnikov, D Pasquier et al. 2D transition metal dichalcogenides. Nat Rev Mater, 2, 17033(2017).
[41] W Luo, M J Zhu, G Peng et al. Carrier modulation of ambipolar few-layer MoTe2 transistors by MgO surface charge transfer doping. Adv Mater, 28, 1704539(2018).
[42] A Avsar, K Marinov, E G Marin et al. Reconfgurable diodes based on vertical WSe2 transistors with van der Waals bonded contacts. Adv Mater, 30, 17072000(2018).
[43] S Kim, J Maassen, J Lee et al. Interstitial Mo-assisted photovoltaic effect in multilayer MoSe2 phototransistors. Adv Mater, 30, 1705542(2018).
[44] S H Song, M K Joo, M Neumann et al. Probing defect dynamics in monolayer MoS2 via noise nanospectroscopy. Nat Commun, 8, 2121(2017).
[45] H Tian, Q S Guo, Y J Xie et al. Anisotropic black phosphorus synaptic device for neuromorphic applications. Adv Mater, 28, 4991(2016).
[46] D Jena, K Banerjee, G H Xing et al. 2D crystal semiconductors: Intimate contacts. Nat Mater, 13, 2640(2014).
[47] L P Xu, P Zhang, H N Jiang et al. Large-scale growth and field-effect transistors electrical engineering of atomic-layer SnS2. Small, 15, 1904116(2019).
[48] G H Han, D L Duong, D H Keum et al. Van der Waals metallic transition metal dichalcogenides. Chem Rev, 118, 6297(2018).
[49] D S Schulman, A J Arnold, S Das. Contact engineering for 2D materials and devices. Chem Soc Rev, 47, 3037(2018).
[50] M Baranowski, A Surrente, L Klopotowski et al. Probing the interlayer exciton physics in a MoS2/MoSe2/MoS2 van der Waals heterostructure. Nano Lett, 17, 6360(2017).
[51] M A Islam, J H Kim, A Schropp et al. Centimeter-scale 2D van der Waals vertical heterostructures integrated on deformable substrates enabled by gold sacrificial layer-assisted growth. Nano Lett, 17, 6157(2017).
[52] C Y Yan, C H Gong, P H Wang et al. 2D group IVB transition metal dichalcogenides. Adv Funct Mater, 28, 1803305(2018).
[53] D Voiry, A Mohite, M Chhowalla. Phase engineering of transition metal dichalcogenides. Chem Soc Rev, 44, 2702(2015).
[54] X S Wang, Z G Song, W Wen et al. Potential 2D materials with phase transitions: structure, synthesis, and device applications. Adv Mater, 31, 1804682(2019).
[55] M S Stark, K L Kuntz, S J Martens et al. Intercalation of layered materials from bulk to 2D. Adv Mater, 31, 1808213(2019).
[56] H Li, S C Ruan, Y J Zeng. Intrinsic van der Waals magnetic materials from bulk to the 2D limit: new frontiers of spintronics. Adv Mater, 31, 1900065(2019).
[57] A Allain, J H Kang, K Banerjee et al. Electrical contacts to two-dimensional semiconductors. Nat Mater, 14, 1195(2015).
[58] J Kang, W Liu, D Sarkar. Computational study of metal contacts to monolayer transitionmetal dichalcogenide semiconductors. Phys Rev X, 4, 031005(2014).
[59] J C Ranuárez, M J Deen, C H Chen. A review of gate tunneling current in MOS devices. Microelectron Reliab, 46, 1939(2016).
[60] Y Liu, J Guo, E B Zhu et al. Approaching the Schottky–Mott limit in van der Waals metal–semiconductor junctions. Nature, 557, 696(2018).
[61] C D English, G Shine, V E Dorgan et al. Improved contacts to MoS2 transistors by ultra-high vacuum metal deposition. Nano Lett, 16, 3824(2016).
[62] K Stokbro, M Engelund, A Blom. Atomicscale model for the contact resistance of the nickelgraphene interface. Phys Rev B, 85, 165442(2012).
[63] I Popov, G Seifert, D Tománek. Designing electrical contacts to MoS2 monolayers: a computational study. Phys Rev Lett, 108, 156802(2012).
[64] W Liu, J H Kang, W Cao et al. High performance few layer MoS2 field-effect-transistor with record low contactresistance. IEEE Int Electron Devices Meet, 19.4. 1(2013).
[65] L Wang, I Meric, P Y Huang et al. One-dimensional electrical contact to a two-dimensional material. Science, 342, 614(2013).
[66] X Cui, G H Lee, Y D Kim et al. Multi-terminal transport measurements of MoS2 using a van der Waals heterostructure device platform. Nat Mater, 10, 534(2015).
[67] Y Chai, R Ionescu, S S Su et al. Making one-dimensional electrical contacts to molybdenum disulfid-based heterostructures through plasma etching. Phys Status Solidi A, 213, 1358(2016).
[68] Y Matsuda, W Q Deng, W A Goddard. Contact resistance for “end-contacted” metal−graphene and metal−nanotube interfaces from quantum mechanics. J Phys Chem C, 114, 17845(2010).
[69] B Karpiak, A Dankert, A W Cummings et al. 1D ferromagnetic edge contacts to 2D graphene/h-BN heterostructures. 2D Mater, 5, 014001(2017).
[70] Y Zhang, L Yin, J W Chu et al. Edge-epitaxial growth of 2D NbS2-WS2 lateral metal-semiconductor heterostructures. Adv Mater, 30, 1803665(2018).
[71] Y J Gong, S D Lei, G L Ye et al. Two-step growth of two-dimensional WSe2/MoSe2 heterostructures. Nano Lett, 15, 6135(2015).
[72] Y G Gong, Z Lin, G L Ye et al. Tellurium-assisted low-temperature synthesis of MoS2 and WS2 monolayers. ACS Nano, 9, 11658(2015).
[73] Q Q Ji, C Li, J L Wang et al. Metallic vanadium disulfide nanosheets as a platform material for multifunctional electrode applications. Nano Lett, 17, 4908(2017).
[74] J D Zhou, J H Lin, X W Huang et al. A library of atomically thin metal chalcogenides. Nature, 556, 358(2018).
[75] W S Leong, Q Q Ji, N N Mao et al. Synthetic lateral metal–semiconductor heterostructures of transition metal disulfides. J Am Chem Soc, 140, 12354(2018).
[76] C S Lee, S J Oh, H Heo et al. Epitaxial van der Waals contacts between transition-metal dichalcogenide monolayer polymorphs. Nano Lett, 19, 1814(2019).
[77] R X Wu, Q Y Tao, W Q Dang et al. van der Waals epitaxial growth of atomically thin 2D metals on dangling-bond-free WSe2 and WS2. Adv Funct Mater, 29, 1806611(2019).
[78] Y Y Jin, Z Y Zeng, Z W Xu et al. Synthesis and transport properties of degenerate p-type Nb-doped WS2 monolayers. Chem Mater, 31, 3534(2019).
[79] J Suh, T E Park, D Y Lin et al. Doping against the native propensity of MoS2: degenerate hole doping by cation substitution. Nano Lett, 14, 6976(2014).
[80] R Kappera, D Voiry, S E Yalcin et al. Phase-engineered low-resistance contacts for ultrathin MoS2 transistors. Nat Mater, 13, 1128(2014).
[81] J Q Zhu, Z G Wang, H Yu et al. Argon plasma induced phase transition in monolayer MoS2. J Am Chem Soc, 139, 10216(2017).
[82] Y J Gong, H T Yuan, C L Wu et al. Spatially controlled doping of two-dimensional SnS2 through intercalation for electronics. Nat Nano, 13, 294(2018).
[83] W Schottky. Zur Halbleitertheorie der sperrschicht-und spitzengleichrichter. Z Phys A, 113, 367(1939).
[84] N Mott. The theory of crystal rectifers. Proc R Soc Lond A, 171, 27(1939).
[85] J Bardeen. Surface states and rectifcation at a metal semi-conductor contact. Phys Rev, 71, 717(1947).
[86] S Das, H Y Chen, A V Penumatcha et al. High performance multi-layer MoS2 transistors with scandium contacts. Nano Lett, 13, 100(2013).
[87] Y Wang, J C Kim, R J Wu et al. Van der Waals contacts between three-dimensional metals and two-dimensional semiconductors. Nature, 568, 70(2019).
[88]
[89] Y Khatami, H Li, C Xu et al. Metal-to-multilayer-graphene contact—Part II: analysis of contact resistance. IEEE Trans Electron Devices, 59, 2453(2012).
[90] Y Khatami, H Li, C Xu et al. Metal-to-multilayer-graphene contact—Part I: contact resistance modeling. IEEE Trans Electron Devices, 59, 2444(2012).
[91] M Zhao, Y Ye, Y Han et al. Large-scale chemical assembly of atomically thin transistors and circuits. Nat Nano, 11, 954(2016).
[92] W Hong, G W Shim, S Y Yang et al. Improved electrical contact properties of MoS2-graphene lateral heterostructure. Adv Funct Mater, 29, 1807550(2019).
[93] W S Leong, C T Nai, J T L Tong. What does annealing do to metal-graphene contacts. Nano Lett, 14, 3840(2014).
[94] F Léonard, A A Talin. Electrical contacts to one- and two-dimensional nanomaterials. Nat Nano, 6, 773(2011).
[95] V Heine. Theory of surface states. Phys Rev, 138, A1689(1965).
[96] L N Liu, J X Wu, L Y Wu et al. Phase-selective synthesis of 1T’ MoS2 monolayers and heterophase bilayers. Nat Mater, 17, 1108(2018).
[97] J Y Zheng, X X Yan, Z X Lu et al. High-mobility multilayered MoS2 flakes with low contact resistance grown by chemical vapor deposition. Adv Mater, 29, 1604540(2017).
[98] C Gong, L Colombo, R M Wallace et al. The unusual mechanism of partial fermi level pinning at metal –MoS2 interfaces. Nano Lett, 14, 1714(2014).
[99] W A Saidi. Trends in the adsorption and growth morphology of metals on the MoS2(001) surface. Cryst Growth Des, 15, 3190(2015).
[100] L J Meng, Y Ma, K P Si et al. Recent advances of phase engineering in group VI transition metal dichalcogenides. Tungsten, 1, 46(2019).
[101] J Li, X D Yang, Y Liu et al. General synthesis of two-dimensional van der Waals heterostructure arrays. Nature, 579, 368(2020).
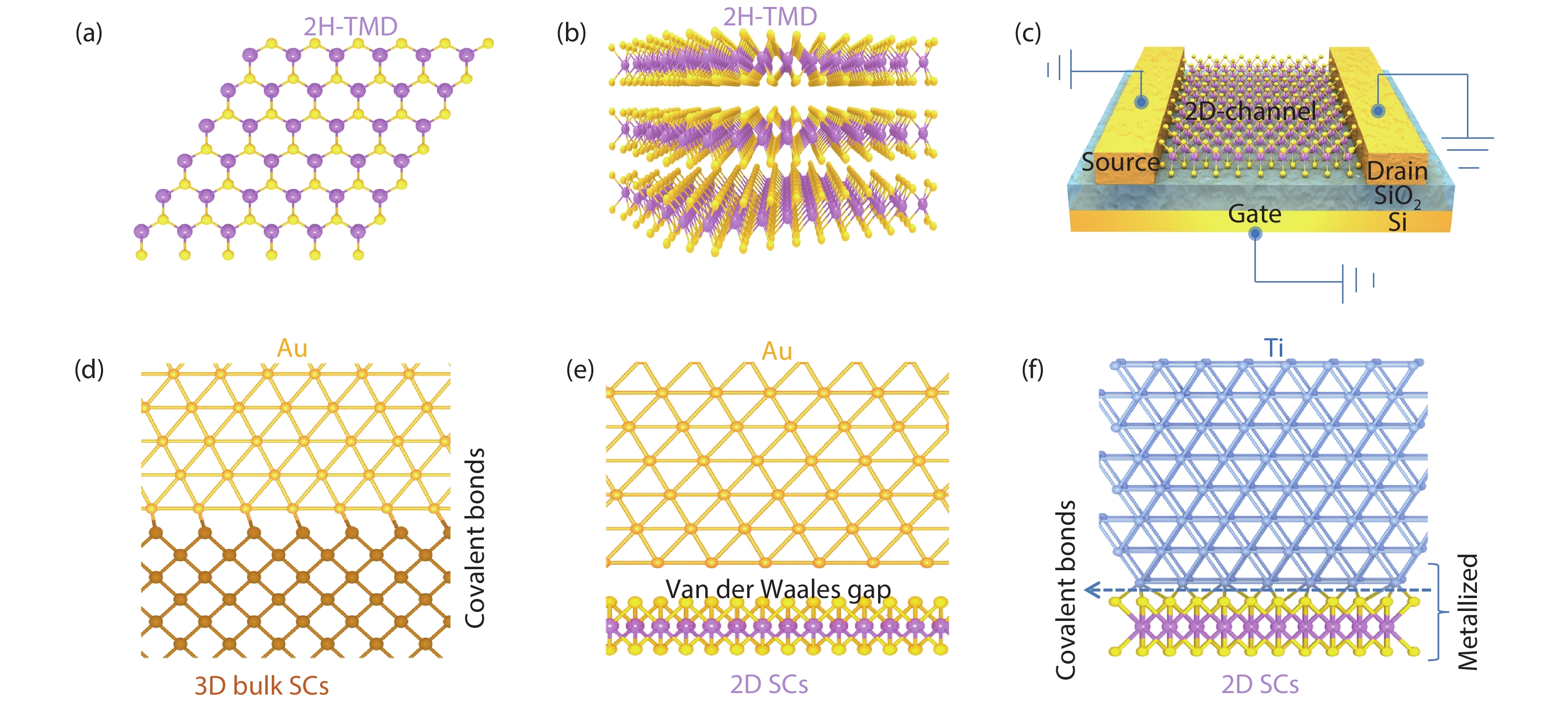
Set citation alerts for the article
Please enter your email address