
- Photonics Research
- Vol. 10, Issue 4, 965 (2022)
Abstract
1. INTRODUCTION
Optical metamaterials as types of emerging materials can alter the temporal and spatial propagation of electromagnetic fields at the nanoscale, resulting in negative refraction [1–5], negative Goos–Hänchen (GH) shift [6,7], anomalous spin Hall effect of light (SHEL) [8,9], super-resolution imaging [10–12], invisibility cloak [13], photoluminescence [14], and other magical functions. The anomalous manipulation of light in metamaterials may bring more attractive and potential applications; the negative GH shift has been proven to achieve slowing down of light [7,15]. However, experimental observation of anomalous SHEL is a real challenge because the spin–orbit interaction is generally weak [16], and its magnitude is exceedingly small. What is more, the development of optical negative index metamaterials has encountered a bottleneck, suffering from extreme propagation losses [17].
In 2015, High
At present, the bottom-up method can realize the preparation of metamaterials without lithography [19]; it has achieved certain results, but the difficulty lies in accurate control of the size and position of the material structure. Superlattices consisting of noble-metal nanoparticles have emerged as a new platform for bottom-up designed metamaterials [20,21] and are expected to generate new negative index materials at visible frequencies. Noble metals are known to interact very efficiently with visible light due to so-called localized surface plasmon resonance (LSPR) excitation; they are highly sensitive to the size, shape, and environment of nanostructures [22]. On this basis, many effective dielectrics have emerged in visible and infrared wave bands using nanoscale metallic particle arrays, but the highly dispersive and lossy metallic response is also troubling [23]. Assembling metallic nanoparticles into macroscopic structures with well-defined order and symmetry is key to achieving material properties [24]. Recently, we found that the unique 3D symmetrical spherical structure of high-generation dendritic macromolecules [25,26] is a powerful tool to assist in the manufacture of metamaterials with uniform size and orderly distribution of metal nanoparticles. Dendritic macromolecules have excellent fractal characteristics. These unique molecular structures and chemical properties are studied in depth and applied in the fields of catalytic reactions [27], biomedicine [28], molecular functionalization [29], preparation of nanomaterials [30], and material modification [31]. Although dendrimers offer remarkable convenience in the field of material preparation, they have never been mentioned for the preparation of metamaterials. Coincidentally, the metal dendritic model [7,9,32,33] has been proven to be a type of metamaterial with multilevel split-ring and equivalent wires. Nanocomposite metamaterials can be obtained using dendrimers to control the particle size and distribution of metal nanoparticles because dendrimers have multilevel-ordered structures similar to the metal dendritic model. This condition is different from highly lossy metallic metamaterials with tightly packed plasma at visible light frequencies [34]; it is highly transparent due to its small particle size and fractal arrangement.
Sign up for Photonics Research TOC. Get the latest issue of Photonics Research delivered right to you!Sign up now
Here, we experimentally demonstrate poly(amidoamine) (PAMAM) dendrimer-based silver nanocomposite metamaterials with abnormal optical response. Using PAMAM as a template and stabilizers, the photochemically reduced Ag can attach to the molecular cavity and grow. Metamaterials PAMAM-Ag prepared by high-generation PAMAM obtain controllable and well-stabilized Ag nanoparticles that are distributed in a multilevel netlike sphere, thereby resulting in anomalous optical manipulation. Experiments show that fifth-generation (5G) PAMAM-Ag can generate a negative GH shift and an anomalous SHEL in visible light broadband (covering the main bands from green light to red light) over a wide range of incident angles; the 5G PAMAM-Ag flat lens [35] shows efficient focusing effect at 750–1050 nm. Finally, a 2D visible light valley–Hall photonic topological insulator (PTI) is simulated based on the dendritic structure of PAMAM-Ag, which may bring more potential applications in topology transmission. The PAMAM-assisted bottom-up preparation method of metamaterials is simple and convenient and provides a new means for developing optical metamaterials.
2. RESULTS AND DISCUSSION
A. Preparation and Characterization
The preparation methods of PAMAM [25,26] have been complete. PAMAM is synthesized through repeated iterative growth; each of its repeated branched layers is called a “generation,” and the synthesis methods include divergent synthesis, convergent synthesis, and the combination of these methods. Dendritic macromolecules have a large number of cavities that can wrap nanoparticles inside, preventing their aggregation and better controlling size, shape, and dispersion. More importantly, dendrimers do not passivate nanoparticles, preserving their optical properties. As shown in Fig. 1(a),
Figure 1.Preparation and characterization of PAMAM-Ag. (a) Schematic of the preparation process of PAMAM-Ag. First, PAMAM and AgNO3 reacted in the dark condition to obtain Ag+ complexed with “N” atom of PAMAM, and then were transferred to UV cold light lamp for photochemical reduction. The blue dots represent Ag nanoparticles. (b)
The film sample is prepared by a spin coating method (1600 r/min for 30 s). The 5G PAMAM-Ag film sample, as an example, has a size of
B. Negative GH Shift and Anomalous SHEL at Visible Frequencies
When a bounded beam is reflected from the interface between two media, it produces small shifts relative to geometric optic prediction. The shift along the interface and in the direction of beam propagation is referred to as GH shift [39], which is described in terms of evanescent wave penetration. Different from GH shift, the SHEL [40] is a result of effective spin–orbit interaction and refers to the transverse splitting in polarizations after reflection or refraction to conserve total angular momentum. These shifts have been used in the field of sensing [41,42]. Furthermore, SHEL can be used to develop spin-dependent devices for precision measurement [43], circular dichroism spectrometric detection [44], and optoelectronic information fields [45]. Some reports focus on enhancing the shift by up to an order of amplification and have made some progress, but they are almost numerical results [46,47]. Current studies have shown that the boundary between the negative index medium and the conventional medium will lead to the reverse anomaly of these shifts, namely, negative GH shift [6,7] and anomalous SHEL [8,9]. Using PAMAM dendrimer-based metamaterials, we experimentally observed clearly identifiable negative GH shift and anomalous SHEL at visible frequencies, covering from the green light wave band to the red light wave band.
5G PAMAM-Ag film samples are measured using the methods proposed by Prajapati et al. [48,49]. Both measurement methods obtain a tiny shift value by analyzing the movement of the interference fringe. GH shift measurement is achieved by the interference of s-polarized and p-polarized beams, and SHEL measurement is achieved by the interference of right-circularly polarized (RCP) and left-circularly polarized (LCP) beams. Figure 2(a) shows a schematic of the experimental setup for GH shift. S-polarized and p-polarized beams from a Wollaston prism are incident on the sample at an angle of
Figure 2.Experimental measurement of the GH shift and SHEL. Schematic of the experimental setup for (a) GH shift and (b) SHEL.
In a previous study, the resonant wave band of the silver dendritic cluster set metasurfaces [7,9] was in the red light band, and the negative GH shift and anomalous SHEL generated by the samples were experimentally observed at 632.8 nm; the hyperbolic metasurface [8] can produce anomalous SHEL at 480–580 nm. Compared with that, PAMAM dendrimer-based silver metamaterials have a wider resonant wave band, which covers at least 532–671 nm in accordance with the experimental results of the negative GH shift and anomalous SHEL. Metamaterial with broadband resonance is conducive to realize the continuous regulation of light, and it contributes to the manufacture of compact and highly integrated devices instead of large volume composite devices.
C. Slab Focusing Effect
A slab focusing experiment [35] is performed to further prove the optical properties of PAMAM-Ag [Fig. 3(a)]. The light beam from the focus of the convex lens reaches the sample after 3–5 mm propagation. The inset shows possible situations (focused or divergent) of transmitted light. The intensity distribution of the transmission light is recorded by a fiber optical spectrometer along the
Figure 3.Slab focusing effect of PAMAM-Ag. (a) Schematic of the experimental setup for flat lens focusing. Inset scenario 1 corresponds to the scene where the flat lens works; scenario 2 occurs when the beams diverge. Light source, LHX150 tungsten light source and monochromator; ND, neutral density filter; FP, fiber probe; FOS, fiber optical spectrometer. (b) Lower panel: measured field amplitude of focused beam generated by 5G PAMAM-Ag film sample at 1050 nm wavelength with the acceptor moving along the
D. Valley–Hall Topological Properties
Furthermore, we proposed a 2D visible light valley–Hall PTI [50] inspired by the multilevel nested dendritic structure of the metamaterial PAMAM-Ag to explore potential applications. As we know, various topological photonic structures with nontrivial bands have been proposed, including coupled ring resonator arrays [51,52], meta-crystals [53], and dielectric photonic crystals [54]. However, existing proposals are mostly limited to macroscale dielectric waveguide structures and complex metamaterials with operation frequency limited to the microwave or infrared bands. The multilevel nested dendritic structure may bring a new design in the field of topological photonics. Especially, it is easy to be directly extended to visible bands with micro-nano scale. The structure of PAMAM-Ag [Fig. 1(a)] was transformed into a hexagonal lattice structure with
Figure 4.Schematic and band structures of the 2D valley–Hall PTI. (a) 3D schematic and top view of the 2D valley–Hall PTI composed of dendritic structure with four-level branch in hexagonal lattice arrangement. Geometric parameters are
According to the 2D irreducible representation of the
3. CONCLUSION
In summary, we have fabricated a visible light metamaterial covering a large area, by using the PAMAM-assisted metallic photochemical reduction approach. The 5G PAMAM-Ag, composed of Ag nanoparticles distributed in a multilevel netlike sphere, is highly transparent in the visible–near infrared wave band and can generate a negative GH shift and anomalous SHEL at resonant frequencies. Focusing experiments confirm that the 5G PAMAM-Ag flat lens focuses effectively at 750–1050 nm, and the maximum focusing light intensity increment reaches 128% compared with the nonfocusing condition. Moreover, the dendritic structures with a three-level branch and four-level branch are proposed to construct 2D valley–Hall PTIs, and the multilevel nest structure can be used for frequency modulation. This anomalous manipulation of light enables metamaterial to possibly be used in subwavelength super-resolution imaging and quantum optical information fields. Simplifying the expensive and time-consuming preparation process of metamaterials, our method merely requires the reduction of silver with the assistance of high-generation PAMAM regardless of the properties of the substrate, providing a new approach for developing larger-scale visible light metamaterials.
APPENDIX A: PREPARATION OF PAMAM
Divergent synthesis is a method to synthesize higher generation products from the center of dendritic macromolecules by extending outward through multiple reaction points. First, ethylenediamine monomer was used as the reaction nucleus to conduct Michael addition reaction with another monomer, methyl acrylate, to obtain half-generation products. Pure half-generation products were obtained by removing excess reactants and by-products through vacuum distillation and extraction methods. Second, the obtained half-generation product was aminated with excess ethylenediamine, and then distillation and extraction were carried out to obtain pure integer-generation dendritic macromolecules. Finally, these steps were repeated to obtain a higher generation of the dendritic PAMAM macromolecule.
APPENDIX B: PREPARATION OF PAMAM-Ag
PAMAM and
APPENDIX C: NUMERICAL SIMULATIONS
The band structures of the unit cells in visible light were calculated by the finite element software COMSOL Multiphysics. The eigenmode propagates in the
References
[1] J. Valentine, S. Zhang, T. Zentgraf, E. Ulin-Avila, D. A. Genov, G. Bartal, X. Zhang. Three-dimensional optical metamaterial with a negative refractive index. Nature, 455, 376-379(2008).
[2] S. P. Burgos, R. de Waele, A. Polman, H. A. Atwater. A single-layer wide-angle negative-index metamaterial at visible frequencies. Nat. Mater., 9, 407-412(2010).
[3] D. Chanda, K. Shigeta, S. Gupta, T. Cain, A. Carlson, A. Mihi, A. J. Baca, G. R. Bogart, P. Braun, J. A. Rogers. Large-area flexible 3D optical negative index metamaterial formed by nanotransfer printing. Nat. Nanotechnol., 6, 402-407(2011).
[4] C. Garcia-Meca, J. Hurtado, J. Marti, A. Martinez, W. Dickson, A. V. Zayats. Low-loss multilayered metamaterial exhibiting a negative index of refraction at visible wavelengths. Phys. Rev. Lett., 106, 067402(2011).
[5] T. Xu, A. Agrawal, M. Abashin, K. J. Chau, H. J. Lezec. All-angle negative refraction and active flat lensing of ultraviolet light. Nature, 497, 470-474(2013).
[6] A. Lakhtakia. Positive and negative Goos-Hänchen shifts and negative phase-velocity mediums (alias left-handed materials). Int. J. Electron. Commun., 58, 229-231(2004).
[7] Z. H. Fang, H. Chen, F. S. Yang, C. R. Luo, X. P. Zhao. Slowing down light using a dendritic cell cluster metasurface waveguide. Sci. Rep., 6, 37856(2016).
[8] A. A. High, R. C. Devlin, A. Dibos, M. Polking, D. S. Wild, J. Perczel, N. P. de Leon, M. D. Lukin, H. Park. Visible-frequency hyperbolic metasurface. Nature, 522, 192-196(2015).
[9] H. Chen, J. Zhao, Z. Fang, D. An, X. Zhao. Visible light metasurfaces assembled by quasiperiodic dendritic cluster sets. Adv. Mater. Interfaces, 6, 1801834(2019).
[10] N. Fang, H. Lee, C. Sun, X. Zhang. Sub-diffraction-limited optical imaging with a silver superlens. Science, 308, 534-537(2005).
[11] J. Rho, Z. Ye, Y. Xiong, X. Yin, Z. Liu, H. Choi, G. Bartal, X. Zhang. Spherical hyperlens for two-dimensional sub-diffractional imaging at visible frequencies. Nat. Commun., 1, 143(2010).
[12] L. Liu, P. Gao, K. Liu, W. Kong, Z. Zhao, M. Pu, C. Wang, X. Luo. Nanofocusing of circularly polarized Bessel-type plasmon polaritons with hyperbolic metamaterials. Mater. Horiz., 4, 290-296(2017).
[13] X. Ni, J. W. Zi, M. Mrejen, Y. Wang, X. Zhang. An ultrathin invisibility skin cloak for visible light. Science, 349, 1310-1314(2015).
[14] L. Y. Beliaev, O. Takayama, P. N. Melentiev, A. V. Lavrinenko. Photoluminescence control by hyperbolic metamaterials and metasurfaces: a review. Opto-Electron. Adv., 4, 210031(2021).
[15] K. L. Tsakmakidis, A. D. Boardman, O. Hess. ‘Trapped rainbow’ storage of light in metamaterials. Nature, 450, 397-401(2007).
[16] O. Hosten, P. Kwiat. Observation of the spin Hall effect of light via weak measurements. Science, 319, 787-790(2008).
[17] V. Shalaev, A. Kildishev, T. Klar, A. Popov, V. Drachev. Optical negative-index metamaterials: from low to no loss. Nat. Photonics, 1, 41-48(2006).
[18] N. Yu, P. Genevet, M. A. Kats, F. Aieta, J. P. Tetienne, F. Capasso, Z. Gaburro. Light propagation with phase discontinuities: generalized laws of reflection and refraction. Science, 334, 333-337(2011).
[19] X. Zhao. Bottom-up fabrication methods of optical metamaterials. J. Mater. Chem., 22, 9439-9449(2012).
[20] K. J. Stebe, E. Lewandowski, M. Ghosh. Materials science. Oriented assembly of metamaterials. Science, 325, 159-160(2009).
[21] H. Alaeian, J. A. Dionne. Plasmon nanoparticle superlattices as optical-frequency magnetic metamaterials. Opt. Express, 20, 15781-15796(2012).
[22] K. L. Kelly, E. Coronado, L. L. Zhao, G. C. Schatz. The optical properties of metal nanoparticles: the influence of size, shape, and dielectric environment. J. Phys. Chem., 107, 668-677(2003).
[23] S. Lee. Colloidal superlattices for unnaturally high-index metamaterials at broadband optical frequencies. Opt. Express, 23, 28170-28181(2015).
[24] K. L. Young, M. B. Ross, M. G. Blaber, M. Rycenga, M. R. Jones, C. Zhang, A. J. Senesi, B. Lee, G. C. Schatz, C. A. Mirkin. Using DNA to design plasmonic metamaterials with tunable optical properties. Adv. Mater., 26, 653-659(2014).
[25] D. A. Tomalia, H. Baker, J. Dewald, M. Hall, G. Kallos, S. Martin, J. Roeck, J. Ryder, P. Smith. A new class of polymers: starburst-dendritic macromolecules. Polym. J., 17, 117-132(1985).
[26] D. A. Tomalia, H. Baker, J. De Wald, M. Hall, G. Kallos, S. Martin, J. Roeck, J. Ryder, P. Smith. Dendritic macromolecules: synthesis of starburst dendrimers. Macromolecules, 19, 2466-2468(1986).
[27] G. Dang, Y. Shi, Z. Fu, W. Yang. Fe3O4@PS@PAMAM-Ag magnetic nanocatalysts and their recoverable catalytic ability. Chin. J. Catal., 33, 651-658(2012).
[28] T. Wang, Y. Zhang, L. Wei, Y. G. Teng, T. Honda, I. Ojima. Design, synthesis, and biological evaluations of asymmetric bow-tie PAMAM dendrimer-based conjugates for tumor-targeted drug delivery. ACS Omega, 3, 3717-3736(2018).
[29] B. Vivek, E. Prasad. Self-assembly-directed aerogel and membrane formation from a magnetic composite: an approach to developing multifunctional materials. ACS Appl. Mater. Interfaces, 9, 7619-7628(2017).
[30] M. A. van Dongen, S. Vaidyanathan, M. M. B. Holl. PAMAM dendrimers as quantized building blocks for novel nanostructures. Soft Matter, 9, 11188-11196(2013).
[31] S. Aliannejadi, A. H. Hassani, H. A. Panahi, S. M. Borghei. Fabrication and characterization of high-branched recyclable PAMAM dendrimer polymers on the modified magnetic nanoparticles for removing naphthalene from aqueous solutions. Microchem. J., 145, 767-777(2019).
[32] X. Zhou, Q. H. Fu, J. Zhao, Y. Yang, X. P. Zhao. Negative permeability and subwavelength focusing of quasi-periodic dendritic cell metamaterials. Opt. Express, 14, 7188-7197(2006).
[33] H. Liu, X. Zhao, Y. Yang, Q. Li, J. Lv. Fabrication of infrared left‐handed metamaterials via double template-assisted electrochemical deposition. Adv. Mater., 20, 2050-2054(2008).
[34] S. J. Palmer, X. Xiao, N. Pazos-Perez, L. Guerrini, M. A. Correa-Duarte, S. A. Maier, R. V. Craster, R. A. Alvarez-Puebla, V. Giannini. Extraordinarily transparent compact metallic metamaterials. Nat. Commun., 10, 2118(2019).
[35] J. B. Pendry. Negative refraction makes a perfect lens. Phys. Rev. Lett., 85, 3966-3969(2000).
[36] K. Esumi, A. Suzuki, N. Aihara, K. Usui, K. Torigoe. Preparation of gold colloids with UV irradiation using dendrimers as stabilizer. Langmuir, 14, 3157-3159(1998).
[37] S. Keki, J. Torok, G. Deak, L. Daroczi, M. Zsuga. Silver nanoparticles by PAMAM-assisted photochemical reduction of Ag+. J. Colloid Interface Sci., 229, 550-553(2000).
[38] U. Kreibig, M. Vollmer. Optical Properties of Metal Clusters(1995).
[39] F. Goos, H. Hanchen. Ein neuer und fundamentaler Versuch zur Totalreflexion. Ann. Phys., 436, 333-346(1947).
[40] M. Onoda, S. Murakami, N. Nagaosa. Hall effect of light. Phys. Rev. Lett., 93, 083901(2004).
[41] S. A. Taya, E. J. El-Farram, T. M. El-Agez. Goos–Hänchen shift as a probe in evanescent slab waveguide sensors. Int. J. Electron. Commun., 66, 204-210(2012).
[42] Y. Yang, T. Lee, M. Kim, C. Jung, T. Badloe, D. Lee, S. Lee, H.-J. Lee, J. Rho. Dynamic optical spin Hall effect in chitosan-coated all-dielectric metamaterials for a biosensing platform. IEEE J. Sel. Top. Quantum Electron., 27, 7300608(2021).
[43] R. Wang, J. Zhou, K. Zeng, S. Chen, X. Ling, W. Shu, H. Luo, S. Wen. Ultrasensitive and real-time detection of chemical reaction rate based on the photonic spin Hall effect. APL Photon., 5, 016105(2020).
[44] A. Shaltout, J. Liu, A. Kildishev, V. Shalaev. Photonic spin Hall effect in gap-plasmon metasurfaces for on-chip chiroptical spectroscopy. Optica, 2, 860-863(2015).
[45] K. Ando, M. Morikawa, T. Trypiniotis, Y. Fujikawa, C. H. W. Barnes, E. Saitoh. Photoinduced inverse spin-Hall effect: conversion of light-polarization information into electric voltage. Appl. Phys. Lett., 96, 082502(2010).
[46] M. Kim, D. Lee, T. H.-Y. Nguyen, H.-J. Lee, G. Byun, J. Rho. Total reflection-induced efficiency enhancement of the spin Hall effect of light. ACS Photon., 8, 2705-2712(2021).
[47] M. Kim, D. Lee, H. Cho, B. Min, J. Rho. Spin Hall effect of light with near-unity efficiency in the microwave. Laser Photon. Rev., 15, 2000393(2020).
[48] C. Prajapati, D. Ranganathan, J. Joseph. Interferometric method to measure the Goos-Hanchen shift. J. Opt. Soc. Am. A, 30, 741-747(2013).
[49] C. Prajapati, D. Ranganathan, J. Joseph. Spin Hall effect of light measured by interferometry. Opt. Lett., 38, 2459-2462(2013).
[50] T. Ma, G. Shvets. All-Si valley-Hall photonic topological insulator. New J. Phys., 18, 025012(2016).
[51] M. Hafezi, E. A. Demler, M. D. Lukin, J. M. Taylor. Robust optical delay lines with topological protection. Nat. Phys., 7, 907-912(2011).
[52] M. Hafezi, S. Mittal, J. Fan, A. Migdall, J. M. Taylor. Imaging topological edge states in silicon photonics. Nat. Photonics, 7, 1001-1005(2013).
[53] A. B. Khanikaev, S. H. Mousavi, W. K. Tse, M. Kargarian, A. H. MacDonald, G. Shvets. Photonic topological insulators. Nat. Mater., 12, 233-239(2013).
[54] L.-H. Wu, X. Hu. Scheme for achieving a topological photonic crystal by using dielectric material. Phys. Rev. Lett., 114, 223901(2015).
[55] D. Malterre, B. Kierren, Y. Fagot-Revurat, C. Didiot, F. G. de Abajo, F. Schiller, J. Cordón, J. Ortega. Symmetry breaking and gap opening in two-dimensional hexagonal lattices. New J. Phys., 13, 013026(2011).
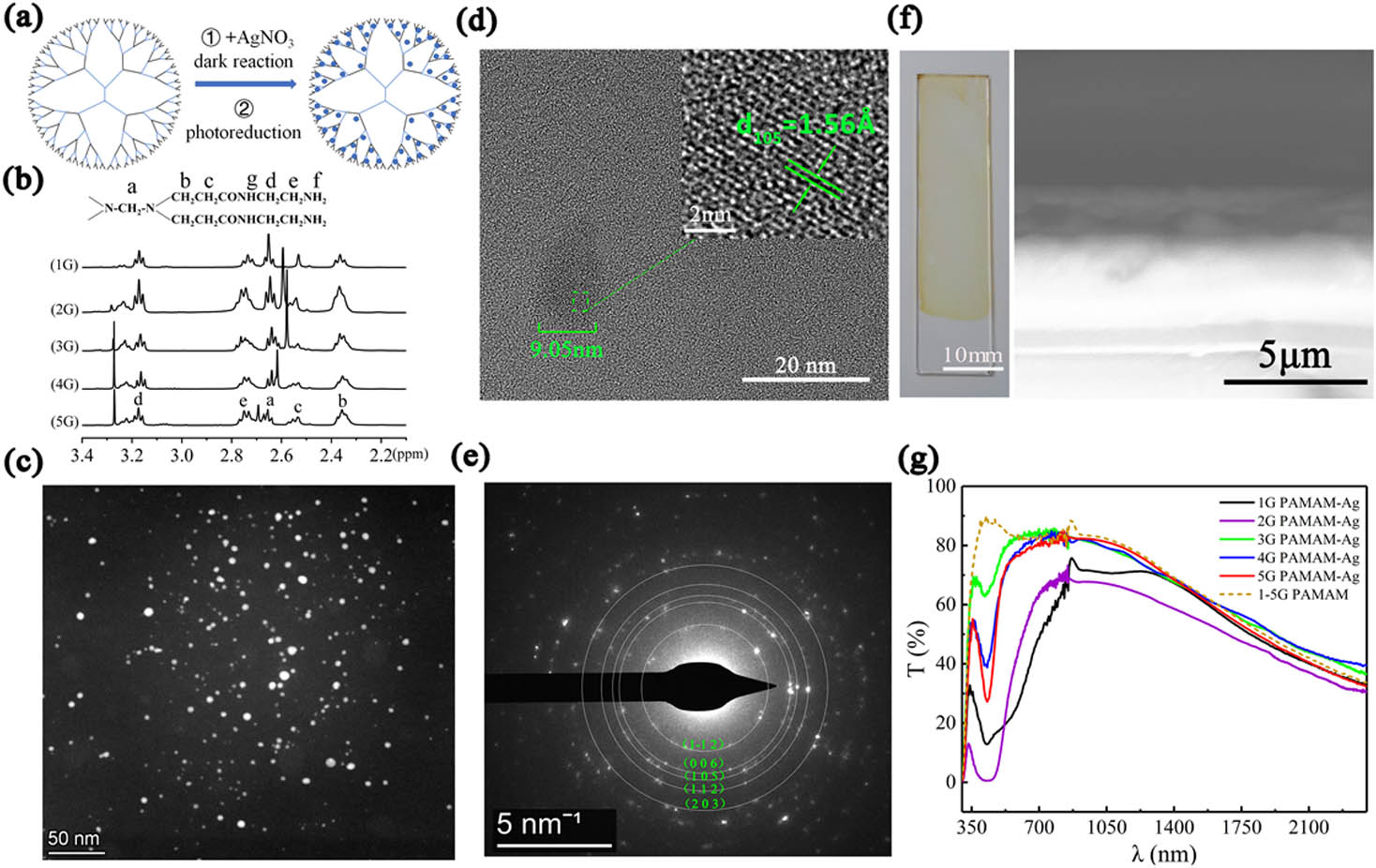
Set citation alerts for the article
Please enter your email address