
- Chinese Optics Letters
- Vol. 19, Issue 9, 092501 (2021)
Abstract
1. Introduction
High-sensitivity ultraviolet (UV) detectors are imperative in many key fields, such as non-line-of-sight communication, missile plume detection, environmental monitoring, corona detection, UV astronomical research, and biological molecule detection[
Along the wide UV wavelength region ranging from 200 to 400 nm, the solar-blind band (240–280 nm) is what people are mostly interested in, which is due to its ultra-low background noise. In literature, although 4H-SiC APDs with both n-i-p and p-i-n structures have been reported with certain success, there is no detailed avalanche mechanism comparison between the two device structures for deep UV detection[
2. Experiment
The SiC APDs are grown on 4 inch (1 inch = 2.54 cm).
Sign up for Chinese Optics Letters TOC. Get the latest issue of Chinese Optics Letters delivered right to you!Sign up now
Figure 1.Schematic cross section structures of the vertical 4H-SiC (a) n-i-p APD and (b) p-i-n APD; (c) top view image of one fabricated APD.
Figure 2.Simulated 2D electric field distribution of a beveled mesa SiC APD under avalanche breakdown voltage. The inset shows the one-dimensional (1D) electric field profile along the black line marked in the 2D electric field profile.
3. Results and Discussion
Figure 3(a) and 3(b) show the room-temperature (RT) I-V and the gain-voltage curves of the SiC n-i-p and p-i-n APDs, respectively. Both devices exhibit a stable dark current of ∼0.1 pA level before avalanche breakdown occurs. The avalanche gain (M) is calculated by the following equation:
Figure 3.I-V and gain-voltage curves of (a) the n-i-p APD and (b) the p-i-n APD; the insets show the enlarged gain regions of the two SiC APDs.
In the spectral response measurement, a high-power xenon lamp is used as the light source and a Horiba iHR320 monochromator is employed to sort monochromatic light. A UV-enhanced Si photodiode (Hamamatsu S1226-8BQ) is used to calibrate the incident light power density. The zero-bias spectral response characteristics of two APDs are plotted in Fig. 4, which exhibit similar shape and magnitude. The peak responsivity of the n-i-p APD is ∼0.114 A/W (QE ∼50%) at 285 nm. Comparatively, the p-i-n APD has a maximum QE of ∼48% at 285 nm. The UV/visible (285 nm/400 nm) rejection ratio of both devices is higher than
Figure 4.Zero-bias spectral response curves of n-i-p and p-i-n APDs.
Next, the single photon detection performance of the n-i-p and p-i-n APDs is compared, which is characterized by a passive quenching circuit [the inset in Fig. 5(a)]. The APDs are biased above
Figure 5.(a) Typical avalanche voltage pulse signal under passive quenching circuit (the inset shows the schematic diagram of passive quenching circuit), (b) the DCR versus normalized overbias curves, (c) the SPDE versus normalized overbias curves, and (d) SPDE versus DCR curves of the n-i-p and p-i-n APDs.
The capacitance of the APDs is calculated by the following equation:
The time taken to bring the APD back to its original state is called the reset time, which is calculated by the following equation:
To understand the detailed avalanche mechanisms of SiC APDs, the photon-induced multiplication characteristics are measured at various wavelengths ranging from 220 nm to 330 nm. Figures 6(a) and 6(b) show the selected gain-voltage curves of the n-i-p and p-i-n APDs obtained under 260 nm, 280 nm, and 300 nm UV illumination, respectively. It is found that with increasing illumination wavelength, the gain-voltage curves of the n-i-p APD shift towards higher voltage with a corresponding higher
Figure 6.Gain-voltage curves measured at 260, 280, and 300 nm illumination for (a) the n-i-p APD and (b) the p-i-n APD. (c) The variation of VB as a function of UV illumination wavelength.
The following physical picture can explain the obvious difference in terms of single photon detection performance and
Figure 7.Schematics of the carrier ionization process at different incident light wavelengths for (a) the n-i-p APD and (b) the p-i-n APD. The illumination wavelength varies from deep UV to near UV wavelength regions.
4. Conclusions
In summary, vertical Geiger mode 4H-SiC n-i-p and p-i-n APDs have been fabricated and characterized. The n-i-p APD exhibits an overall better single photon counting performance for deep UV detection, which can be explained by its dominant hole-initiated avalanche characteristics. Comparatively, the avalanche events in p-i-n APDs are mainly electron-initiations in the deep UV wavelength region. Since the impact ionization coefficient ratio of holes to electrons is much larger than one, 4H-SiC APDs with dominant hole-initiated avalanche would feature relatively lower critical electric field strength and then fewer dark counts. In addition, this is the first time, to the best of our knowledge, to report single photon detection characteristics of vertical SiC n-i-p-n APDs with an enhanced fill factor.
References
[1] H. Chen, K. Liu, L. Hu, A. A. Al-Ghamdi, X. Fang. New concept ultraviolet photodetectors. Mater. Today, 18, 493(2015).
[2] Y. Wang, Y. Qian, X. Kong. Photon counting based on solar-blind ultraviolet intensified complementary metal-oxide-semiconductor (ICMOS) for corona detection. IEEE Photon. J., 10, 7000919(2018).
[3] R. H. Hadfield. Single-photon detectors for optical quantum information applications. Nat. Photon., 3, 696(2009).
[4] X. Yi, S. Xie, B. Liang, L. W. Lim, J. S. Cheong, M. C. Debnath, D. L. Huffaker, C. H. Tan, J. P. R. David. Extremely low excess noise and high sensitivity AlAs0.56Sb0.44 avalanche photodiodes. Nat. Photon., 13, 683(2019).
[5] J. C. Campbell. Recent advances in avalanche photodiodes. J. Lightwave Technol., 34, 278(2016).
[6] C. J. Chunnilall, I. P. Degiovanni, S. Kueck, I. Müller, A. G. Sinclair. Metrology of single-photon sources and detectors: a review. Opt. Eng., 53, 1910(2014).
[7] A. Vert, S. Soloviev, P. Sandvik. SiC avalanche photodiodes and photomultipliers for ultraviolet and solar-blind light detection. Phys. Status Solidi A, 206, 2468(2009).
[8] A. R. Powell, L. B. Rowland. SiC materials-progress, status, and potential roadblocks. Proc. IEEE, 90, 942(2002).
[9] X. Bai, H. Liu, D. C. Mcintosh, J. C. Campbell. High-detectivity and high-single-photon-detection-efficiency 4H-SiC avalanche photodiodes. IEEE J. Quantum Electron., 45, 300(2009).
[10] D. Zhou, F. Liu, H. Lu, D. Chen, F. Ren, R. Zhang, Y. Zheng. High-temperature single photon detection performance of 4H-SiC avalanche photodiodes. IEEE Photon. Technol. Lett, 26, 1136(2014).
[11] X. Guo, A. L. Beck, Z. Huang, N. Duan, J. C. Campbell, D. Emerson, J. J. Sumakeris. Performance of low-dark-current 4H-SiC avalanche photodiodes with thin multiplication layer. IEEE Trans. Electron Devices, 53, 2259(2006).
[12] J. Hu, X. Xin, X. Li, J. H. Zhao, B. L. VanMil, K. K. Lew, R. L. Myers-Ward, C. R. Eddy, D. K. Gaskill. 4H-SiC visible-blind single-photon avalanche diode for ultraviolet detection at 280 and 350 nm. IEEE Trans. Electron Devices, 55, 1977(2008).
[13] X. Y. Zhou, J. Li, W. L. Lu, Y. Wang, X. Song, S. Yin, X. Tan, Y. Lü, H. Guo, G. Gu, Z. Feng. Large-area 4H-SiC avalanche photodiodes with high gain and low dark current for visible-blind ultraviolet detection. Chin. Opt. Lett., 16, 060401(2018).
[14] X. Y. Zhou, X. Tan, Y. Wang, X. Song, T. Han, J. Li, W. Lu, G. Gu, S. Liang, Y. Lü, Z. Feng. High-performance 4H-SiC p-i-n ultraviolet avalanche photodiodes with large active area. Chin. Opt. Lett., 17, 090401(2019).
[15] A. L. Beck, B. Yang, X. Guo, J. C. Campbell. Edge breakdown in 4H-SiC avalanche photodiodes. IEEE J. Quantum Electron., 40, 321(2004).
[16] X. Guo, A. L. Beck, J. C. Campbell, D. Emerson, J. Sumakeris. Spatial nonuniformity of 4H-SiC avalanche photodiodes at high gain. IEEE J. Quantum Electron., 41, 1213(2005).
[17] X. Cai, L. Li, H. Lu, D. Zhou, W. Xu, D. Chen, F. Ren, R. Zhang, Y. Zheng, G. Li. Vertical 4H-SiC n-i-p-n APDs with partial trench isolation. IEEE Photon. Technol. Lett, 30, 805(2018).
[18] S. Yang, D. Zhou, X. Cai, W. Xu, H. Lu, D. Chen, F. Ren, R. Zhang, Y. Zheng, R. Wang. Analysis of dark count mechanisms of 4H-SiC ultraviolet avalanche photodiodes working in Geiger mode. IEEE Trans. Electron Devices, 64, 4532(2017).
[19] H. Y. Cha, P. M. Sandvik. Electrical and optical modeling of 4H-SiC avalanche photodiodes. Jpn. J. Appl. Phys., 47, 5423(2008).
[20] T. Hatakeyama, T. Watanabe, T. Shinohe, K. Kojima, K. Arai, N. Sano. Impact ionization coefficients of 4H silicon carbide. Appl. Phys. Lett., 85, 1380(2004).
[21] J. E. Green, W. S. Loh, A. R. J. Marshall, R. C. Tozer, J. P. R. David, S. I. Soloviev, P. M. Sandvik. Impact ionization coefficients in 4H-SiC by ultralow excess noise measurement. IEEE Trans. Electron Devices, 59, 1030(2012).
[22] M. Hjelm, H. Nilsson, A. Martinez, K. F. Brennan, E. Bellotti. Monte Carlo study of high-field carrier transport in 4H-SiC including band-to-band tunneling. J. Appl. Phys., 93, 1099(2003).
[23] H. Niwa. Impact ionization coefficients in 4H-SiC toward ultrahigh-voltage power devices. IEEE Trans. Electron Devices, 62, 3326(2015).
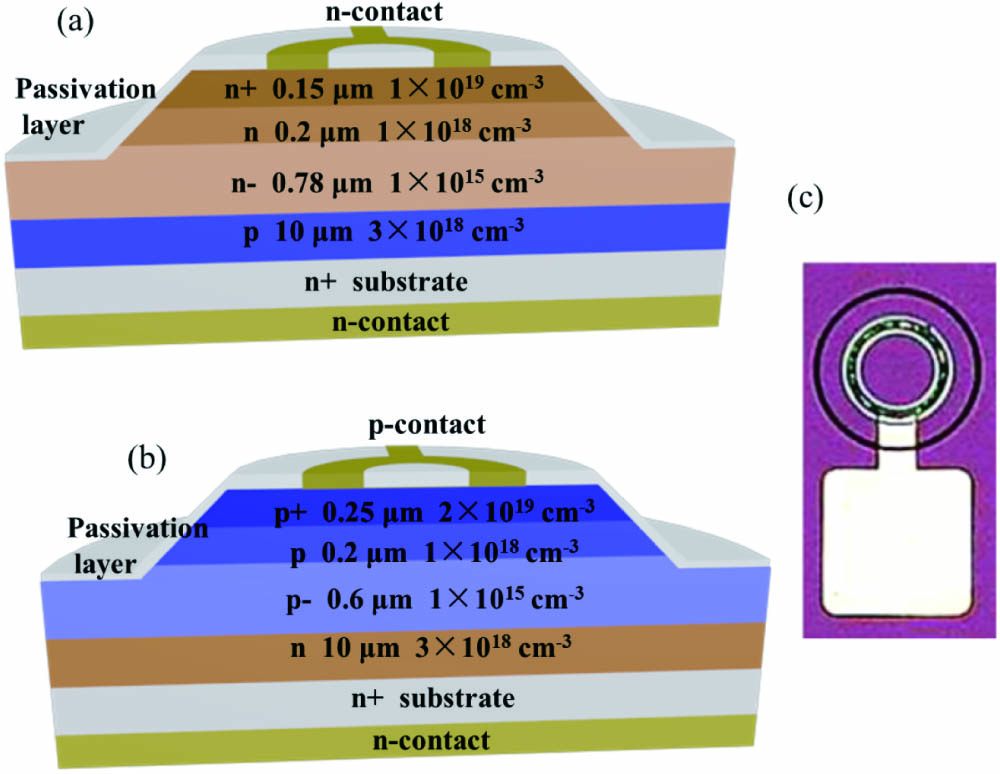
Set citation alerts for the article
Please enter your email address