
- Opto-Electronic Advances
- Vol. 4, Issue 8, 200063-1 (2021)
Abstract
Introduction
The rampant appearance of counterfeit products has caused tremendous economic losses to customers and copyright owners, and the phenomena from fake medicines to fake military equipment have made it clear that the counterfeit business has become an economic, human health and national security issue
As a novel type of multifunctional luminescent materials, metal-organic frameworks (MOFs), constructed by metal ions/clusters and organic ligands through coordinate bonds, have received tremendous attention due to their predicable structures, high porosity, and easily tailorable functions
Considering that chemical anti-counterfeiting methods can be an efficient way for the encryption of information, herein, we present to utilize the multiple centers of MOFs to develop multicolor luminescence under simply external stimuli by precisely designing the synergy emission between metal ions and ligands. The introduction of functional groups as guest interactive sites can interact with external stimuli (the key to analyze information) on the organic ligand, and change the nonradiative decay pathways and energy transfer of MOFs, affecting the final luminescent color. As a proof-of-concept experiment, we design to use the most commonly used water in nature as the key to change the luminescent state of multiple emission centers of a lanthanide MOF, Eu(BDC-NH2) (BDC-NH2 = 2-aminoterephthalate), and thus obtain multicolor luminescence. Benefiting from the suitable stability of Eu(BDC-NH2) in water and hydrogen-bond interaction between -NH2 group and water molecule, as-synthesized Eu(BDC-NH2) exhibits gradually enhanced ligand-based luminescence and quenched lanthanide emission with the increasing the water content. And, crucially, the inverse change of emission intensity from ligands and lanthanide ions enables Eu(BDC-NH2) to generate colorful luminescence under different water content, and the specific content of water can be used as the key to add the level of encryption for the anti-counterfeiting ink. Meanwhile, the variable luminescence colors that are visible to the naked eye guarantee the feasibility of Eu(BDC-NH2) for in situ visualization of the multicolor information.
Experimental section
Synthesis of Eu(BDC-NH2), Tb(BDC-NH2) and Gd(BDC-NH2): Eu(BDC-NH2) was synthesized by the solvothermal method. A mixture of Eu(NO3)3·6H2O (323.4 mg, 0.725 mmol), 2-aminoterephthalic acid (H2BDC-NH2, 131.3 mg, 0.725 mmol), 2-fluorobenzoic acid (2-FBA, 812.6 mg, 5.8 mmol), DMF (36.5 mL), H2O (3 mL), and HNO3 (2 mL, 3.5 M in DMF) was added to a 100 mL Teflon-lined stainless steel reactor, heated at 110 °C for 60 h, and then slowly cooled to room temperature. The colorless crystals of Eu(BDC-NH2) were collected and washed with dimethylformamide (DMF). Elemental analysis: Anal. Calcd. for (Me2NH2)2[Eu6(μ3-OH)8(BDC-NH2)6(H2O)6]·5DMF (C67H101Eu6N13O43, 2688.39): C 29.93, H 3.76, N 6.76; found: C 30.56, H 3.72, N 6.67. Tb(BDC-NH2) and Gd(BDC-NH2) were synthesized similarly to Eu(BDC-NH2) by using Tb(NO3)3·6H2O and Gd(NO3)3·6H2O instead of Eu(NO3)3·6H2O, respectively. X-ray crystal data for Eu(BDC-NH2) show: C67H101Eu6N13O43, Mw = 2688.39, 0.2 × 0.2 × 0.2 mm3, Cubic,
[CCDC 1500928 contains the supplementary crystallographic data for this paper. These data can be obtained free of charge from The Cambridge Crystallographic Data Center via www.ccdc.cam.ac.uk/data_request/cif.]
Results and discussion
The MOF Eu(BDC-NH2) was synthesized through the solvothermal reaction between Eu(NO3)3·6H2O and 2-aminoterephthalic acid (H2BDC-NH2) in mixed solvents of N, N-DMF and water using 2-fuorobenzoic acid (2-FBA) as a cluster directing agent according to the method reported by Eddaoudi et al
Figure 1.(
Single-crystal X-ray diffraction analysis indicates that Eu(BDC-NH2) crystallizes in the cubic
The stability of Eu(BDC-NH2) in water was evaluated by testing the amount of dissolved ligand in supernatant of Eu(BDC-NH2) aqueous suspension (10 mL, 10 mg·mL−1) with the elapse of time by UV absorption spectrum. As shown in Fig. 2(a), with the increase of soaking time, the amount of dissolved ligand increased rapidly at the beginning, and the increase rate slowed down after 1 day, and then reached the equilibrium after about 3 days with the equilibrium concentration about 5.51×10−4 mol·L−1. According to the molecular formula of Eu(BDC-NH2), the dissolved Eu(BDC-NH2) was calculated to be 2.50% of the original. More interestingly, the undissolved portion of Eu(BDC-NH2) can maintain its framework and porosity intact. The measured PXRD patterns of Eu(BDC-NH2) soaked in water for 48 h at room temperature show retained crystallinity and unchanged structures ( Fig. S5, Supplementary information). This retention of framework and porosity in water was further authenticated by the preservation of the original apparent BET surface area with a slight decrease, as evidenced by the conserved characteristics of the nitrogen adsorption isotherm (Fig. 2(b)). All of the above results indicate a suitable stability of aqueous Eu(BDC-NH2) suspension at room temperature.
Figure 2.(
The excitation spectra of Eu(BDC-NH2) displays an intense and broad band with a maximum at around 375 nm, which is ascribed to the π-π* electron transition of BDC-NH22− ligands ( Figs. S6−S9, Supplementary information). Upon excited at 375 nm, the solid sample of Eu(BDC-NH2) exhibits characteristic emission peaks of Eu3+ ions at 579, 594, 614, 653, and 700 nm, which can be ascribed to the 5D0 → 7FJ (J = 0, 1, 2, 3 and 4) transitions, respectively (Fig. 3(a)). It is worth noting that no apparent residual emission from the BDC-NH22− ligand was observed in solid-state Eu(BDC-NH2), indicating that the excitation energy absorbed by the ligands is efficiently transferred to the Eu3+ ions. Similarly, the suspension of Eu(BDC-NH2) in THF exhibits the strong characteristic emission peaks of Eu3+ ions (Fig. 3(b)). However, the characteristic 4f-4f transitions of Eu3+ ions are almost totally quenched while the ligand-based luminescence centered at 430 nm is drastically enhanced when Eu(BDC-NH2) is suspended in water (Fig. 3(c)).
Figure 3.Emission spectra of Eu(BDC-NH2) in the solid state (
Encouraged by the changed luminescence of Eu(BDC-NH2) under water treatment, we sought to explore its potential for multicolor luminescence through tuning the content of water. As a proof-of-concept experiment, the in situ emission spectra were monitored with the piece by piece addition of water in 2 mL of dry organic solvents (THF, ethanol, acetone, and acetonitrile) where Eu(BDC-NH2) (1 mg) was suspended. As shown in Fig. 4(a, b), the luminescence intensity of BDC-NH22− ligands at 430 nm substantially increases with the addition of water in THF from 0 to 5 vol%, concomitant with a dramatic decrease of the emissions of Eu3+ ions when excited at 375 nm. The luminescence intensity ratio between ligand (430 nm) and Eu3+ ion (614 nm) in Eu(BDC-NH2) can be correlated well with the water content in THF by a linearly relationship of
Figure 4.(
with correlation coefficient 0.9975 over a wide range from 0 to 5 vol% (Fig. 4(c)), where I430 and I614 are the luminescence intensity of ligand and Eu3+, respectively; c is the water content in THF (vol%). As shown in Fig. S10 (Supplementary information), Eu(BDC-NH2) can work even in the ultra-low water content range such as 0–0.1 vol% and 0–1 vol%. Similar trends of emission spectral changes and linear calibration curves were also observed upon adding water to the suspension of Eu(BDC-NH2) in ethanol, acetone, and acetonitrile ( Figs. S11, S12 and S13, Supplementary information). In addition, the fluorescence titrations were also performed using 0.5 and 1.5 mg of Eu(BDC-NH2) as fluorescent indicator, respectively. The results indicate that the luminescence intensity ratio (I430/I614) only depends on the water content while independent of the amount of Eu(BDC-NH2) used for multicolor luminescence (Fig. 4(d)), thus demonstrating that Eu(BDC-NH2) is an excellent anti-counterfeiting matrix and does not require additional processing steps for reading out multicolor information. To further demonstrate the versatility of this strategy, similar experiments were also performed using the isostructural MOF Tb(BDC-NH2) as a sensor. As expected, the luminescence intensity ratio between the ligand and Tb3+ is also linearly dependent on the water content from 0 to 5 vol% in THF ( Fig. S14, Supplementary information).
The multicolor luminescence of designed MOFs Eu(BDC-NH2) under external water treatment for multi-level information anti-counterfeiting is feasible, but the stability of Eu(BDC-NH2) is also necessary because the anti-counterfeiting tags on the product always face various types of environment. Therefore, it is worth mentioning that the photostability of Eu(BDC-NH2) is especially outstanding ( Fig. S15, Supplementary information) and the fluorescent response toward water is immediate. As is shown in Fig. S16, it takes just 12 s of Eu3+ emission to accomplish 95% of the overall luminescent intensity variation, and ligand luminescence increases steeply after the addition of water, suggesting the very fast and real-time responsive ability of Eu(BDC-NH2). More importantly, the responsive ability of Eu(BDC-NH2) can be easily recovered and regenerated by simply removing the dissolved part with organic solvents. As shown by the repeatability experiment ( Fig. S17, Supplementary information) and PXRD ( Fig. S18, Supplementary information), the frameworks of Eu(BDC-NH2) are also intact and the luminescent intensity ratio is fully reversible without significant hysteresis after four consecutive of detection cycles.
Due to the different luminescent colors and opposite intensity changes of ligand and Eu3+ ion, the Eu(BDC-NH2) sensor exhibits a prominent luminescence color change, which is almost linearly tuned from red to blue with the addition of water in THF. Figure 5(a) shows the emission colors of Eu(BDC-NH2) in Commission Internacionale de L’Eclairage (CIE) chromaticity diagram transformed from the corresponding water-dependent emission spectra, which changes from (0.562, 0.295) to (0.184, 0.080) when the water content increases from 0 vol% to 5 vol%. It can be found that the color evolution strongly depends on the water content and even a slight increase of the water content can cause obvious color change in THF and EtOH (Fig. 5(b, c)). Especially, different water content is used as a key to read anti-counterfeiting information under specific color, which greatly improves the security of anti-counterfeiting materials. Meanwhile, such a significant color switching can be easily observed by the naked eye and captured by a single CCD camera, thus enabling us to directly visualize the water content in various organic solvents. Above results demonstrate that Eu(BDC-NH2) is an excellent candidate for anti-counterfeiting materials with real-time, robust and reusable multicolor luminescence by simple water treatment, thus providing a significant advantage over the traditional anti-counterfeiting tags.
Figure 5.(
Such a highly sensitive luminescence response of Ln(BDC-NH2) toward water is mainly due to the following three reasons: 1) hydrogen-bond interaction between -NH2 group and water molecules; 2) quenching of Ln3+ emission by the effect of O-H oscillators, which is universal and acknowledged and 3) the changed energy transfer process of Ln(BDC-NH2).
Among these reasons, the quenching of Ln3+ emission caused by O-H oscillators is the dominant one. This is because BDC-NH2 possesses hydrophilic active sites -NH2, thus water molecules enter cages easily, and meanwhile, the introduction of water results in the increase of nonradiative decay pathways (O-H oscillators) that prevents radiative relaxation (emission), accounting for the quenching of Ln3+ emission
Figure 6.(
Conclusion
In summary, we have successfully illustrated a proof of concept for real-time and multicolor luminescence under simple water treatment in organic solvents using a lanthanide MOF Eu(BDC-NH2). Due to the unique responsive mechanism based on increased nonradiative decay pathways and Eu3+-to-ligand energy back transfer process, the ligand-based luminescence in Eu(BDC-NH2) significantly enhanced while the Eu3+ emission decreased with the addition of water content. Accordingly, the synergistic luminescence of Eu(BDC-NH2) from ligand and Eu3+ emissive centers exhibits a broad span range in CIE chromaticity coordinates, resulting in multicolor luminescence in visible range. More importantly, the different emission colors obtained by water treatment correspond to specific water content, which can be used as an encryption key for high-level anti-counterfeiting tags. Meanwhile, the variable luminescence color makes Eu(BDC-NH2) have excellent potential for in situ visualizing the multicolor information in organic solvents straightforwardly. The strategy we report here would open a new avenue to develop multicolor luminescent MOFs under simple external stimuli for high-level anti-counterfeiting and stimulate more applied research.
References
[1] W Ren, GG Lin, C Clarke, JJ Zhou, DY Jin. Optical nanomaterials and enabling technologies for high-security-level anticounterfeiting. Adv Mater, 32, 1901430(2020).
[2] T Staake, F Thiesse, E Fleisch. The emergence of counterfeit trade: a literature review. Eur J Mark, 43, 320-349(2009).
[3] RM Li, YT Zhang, J Tan, JX Wan, J Guo et al. Dual-mode encoded magnetic composite microsphere based on fluorescence reporters and raman probes as covert tag for anticounterfeiting applications. ACS Appl Mater Interfaces, 8, 9384-9394(2016).
[4] EL Prime, DH Solomon. Australia’s plastic banknotes: fighting counterfeit currency. Angew Chem Int Ed, 49, 3726-3736(2010).
[5] WJ Yao, QY Tian, W Wu. Tunable emissions of upconversion fluorescence for security applications. Adv Opt Mater, 7, 1801171(2019).
[6] XF Ji, RT Wu, LL Long, XS Ke, CX Guo et al. Encoding, reading, and transforming information using multifluorescent supramolecular polymeric hydrogels. Adv Mater, 30, 1705480(2018).
[7] R Arppe, TJ Sørensen. Physical unclonable functions generated through chemical methods for anti-counterfeiting. Nat Rev Chem, 1, 0031(2017).
[8] C Zhang, L Yang, J Zhao, BH Liu, MY Han et al. White‐light emission from an integrated upconversion nanostructure: toward multicolor displays modulated by laser power. Angew Chem Int Ed, 54, 11531-11535(2015).
[9] YQ Lu, JB Zhao, R Zhang, YJ Liu, DM Liu et al. Tunable lifetime multiplexing using luminescent nanocrystals. Nat Photonics, 8, 32-36(2014).
[10] GY Chen, J Damasco, HL Qiu, W Shao, TY Ohulchanskyy et al. Energy-cascaded upconversion in an organic dye-sensitized core/shell fluoride nanocrystal. Nano Lett, 15, 7400-7407(2015).
[11] JJ Zhou, SH Wen, JY Liao, C Clarke, SA Tawfik et al. Activation of the surface dark-layer to enhance upconversion in a thermal field. Nat Photonics, 12, 154-158(2018).
[12] JC Zhang, C Pan, YF Zhu, LZ Zhao, HW He et al. Achieving thermo-mechano-opto-responsive bitemporal colorful luminescence via multiplexing of dual lanthanides in piezoelectric particles and its multidimensional anticounterfeiting. Adv Mater, 30, 1804644(2018).
[13] GR Cai, HL Jiang. A modulator-induced defect-formation strategy to hierarchically porous metal-organic frameworks with high stability. Angew Chem Int Ed, 56, 563-567(2017).
[14] ZJ Chen, PH Li, R Anderson, XJ Wang, X Zhang et al. Balancing volumetric and gravimetric uptake in highly porous materials for clean energy. Science, 368, 297-303(2020).
[15] T Islamoglu, ZJ Chen, MC Wasson, CT Buru, KO Kirlikovali et al. Metal-organic frameworks against toxic chemicals. Chem Rev, 120, 8130-8160(2020).
[16] S Lee, EA Kapustin, OM Yaghi. Coordinative alignment of molecules in chiral metal-organic frameworks. Science, 353, 808-811(2016).
[17] J Li, XX Wang, GX Zhao, CL Chen, ZF Chai et al. Metal-organic framework-based materials: superior adsorbents for the capture of toxic and radioactive metal ions. Chem Soc Rev, 47, 2322-2356(2018).
[18] P Li, NA Vermeulen, CD Malliakas, DA Gómez-Gualdrón, AJ Howarth et al. Bottom-up construction of a superstructure in a porous uranium-organic crystal. Science, 356, 624-627(2017).
[19] B Wang, X Zhang, HL Huang, ZJ Zhang, T Yildirim et al. A microporous aluminum-based metal-organic framework for high methane, hydrogen, and carbon dioxide storage. Nano Res, 14, 507-511(2021).
[20] BX Yu, G Ye, J Chen, SQ Ma. Membrane-supported 1D MOF hollow superstructure array prepared by polydopamine-regulated contra-diffusion synthesis for uranium entrapment. Environ Pollut, 253, 39-48(2019).
[21] X Zhang, RB Lin, J Wang, B Wang, B Liang et al. Optimization of the pore structures of MOFs for record high hydrogen volumetric working capacity. Adv Mater, 32, 1907995(2020).
[22] HY Yuan, JF Tao, NX Li, A Karmakar, CH Tang et al. On‐chip tailorability of capacitive gas sensors integrated with metal-organic framework films. Angew Chem Int Ed, 58, 14089-14094(2019).
[23] YN Yao, ZH Gao, YC Lv, XQ Lin, YY Liu et al. Heteroepitaxial growth of multiblock Ln-MOF microrods for photonic barcodes. Angew Chem Int Ed, 58, 13803-13807(2019).
[24] H Kim, S Yang, SR Rao, S Narayanan, EA Kapustin et al. Water harvesting from air with metal-organic frameworks powered by natural sunlight. Science, 356, 430-434(2017).
[25] HL Nguyen, N Hanikel, SJ Lyle, CH Zhu, DM Proserpio et al. A porous covalent organic framework with voided square grid topology for atmospheric water harvesting. J Am Chem Soc, 142, 2218-2221(2020).
[26] D Ma, P Li, XY Duan, JZ Li, PP Shao et al. A hydrolytically stable vanadium(IV) metal-organic framework with photocatalytic bacteriostatic activity for autonomous indoor humidity control. Angew Chem Int Ed, 59, 3905-3909(2020).
[27] NC Burtch, H Jasuja, KS Walton. Water stability and adsorption in metal-organic frameworks. Chem Rev, 114, 10575-10612(2014).
[28] JN Hao, YS Li. Concurrent modulation of competitive mechanisms to design stimuli-responsive Ln-MOFs: a light-operated dual-mode assay for oxidative DNA damage. Adv Funct Mater, 29, 1903058(2019).
[29] NS Zhao, LJ Li, XZ Song, M Zhu, ZM Hao et al. Lanthanide ion codoped emitters for tailoring emission trajectory and temperature sensing. Adv Funct Mater, 25, 1463-1469(2015).
[30] ZQ Li, GN Wang, YX Ye, B Li, HR Li et al. Loading photochromic molecules into a luminescent metal-organic framework for information anticounterfeiting. Angew Chem Int Edit, 58, 18025-18031(2019).
[31] SAA Razavi, A Morsali. Linker functionalized metal-organic frameworks. Coordin Chem Rev, 388, 213023(2019).
[32] V Guillerm, ŁJ Weseliński, Y Belmabkhout, AJ Cairns, V D'Elia et al. Discovery and introduction of a (3, 18)-connected net as an ideal blueprint for the design of metal-organic frameworks. Nat Chem, 6, 673-680(2014).
[33] DX Xue, Y Belmabkhout, O Shekhah, H Jiang, K Adil et al. Tunable rare earth fcu-MOF platform: access to adsorption kinetics driven gas/vapor separations via pore size contraction. J Am Chem Soc, 137, 5034-5040(2015).
[34] Y Yu, JP Ma, YB Dong. Luminescent humidity sensors based on porous Ln3+-MOFs. CrystEngComm, 14, 7157-7160(2012).
[35] L Yu, QT Zheng, H Wang, CX Liu, XQ Huang et al. Double-color lanthanide metal-organic framework based logic device and visual ratiometric fluorescence water microsensor for solid pharmaceuticals. Anal Chem, 92, 1402-1408(2020).
[36] L Li, YL Zhu, XH Zhou, CDS Brites, D Ananias et al. Visible‐light excited luminescent thermometer based on single lanthanide organic frameworks. Adv Funct Mater, 26, 8677-8684(2016).
[37] J Heine, K Müller-Buschbaum. Engineering metal-based luminescence in coordination polymers and metal-organic frameworks. Chem Soc Rev, 42, 9232-9242(2013).
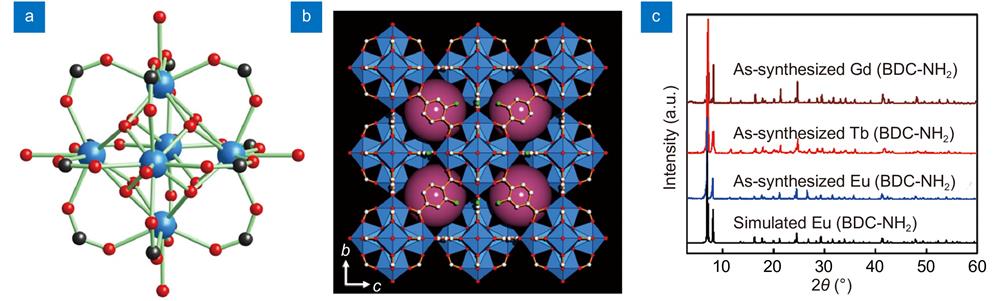
Set citation alerts for the article
Please enter your email address