
- Photonics Research
- Vol. 9, Issue 11, 2144 (2021)
Abstract
1. INTRODUCTION
Metallic particles are drawing increasing attention for their size- and shape-dependent optical properties [1], which have made them very effective tools in various applications, acting, for example, as drug carriers in drug delivery [2], label-free biosensors in biosensing [3], and optical nano-antennas in optical communications [4]. They have also been exploited as remotely, optically controlled micro/nanoscopic sources of heat for bubble formation [5], surface ejection [6], and cancer therapy [7]. Among these applications, efficient manipulation of metallic particles is of essential importance. Optical tweezers, a technique that uses focused light to manipulate microparticles, provide an elegant means.
Compared with dielectric nanoparticles, metallic nanoparticles in the Rayleigh size regime are better candidates for optical trapping due to their higher polarizability [8,9]. Numerous theoretical and experimental works during the past two decades have shown that metallic nanoparticles exhibit higher trapping efficiency than their dielectric counterparts due to much larger gradient force while experiencing negligible scattering force [10–15]. However, as the particles’ size increases, the scattering force increases dramatically due to their high extinction coefficient, making large-size metallic particles hard to trap. Theoretical work has been carried out to find the solutions to stable three-dimensional (3D) trapping of such particles [16–20]. Early research revealed that Gaussian beam could provide stable two-dimensional trapping of gold Mie particles in the transverse plane [16,17]. Further simulations by Gu
This paper reports a new mechanism of stable 3D confinement of metallic (gold) microparticles using a linearly polarized Gaussian beam. Numerical calculations show that the metallic (gold) microparticle can be stably confined at two off-axis positions in the vicinity of the focal plane by the vortex-like force field. This confinement is different from the optical levitation on the optical axis as reported previously [20]. Furthermore, the off-axis levitated gold microparticle will execute transverse spinning due to the torque arising from the asymmetric force field. Generally, optical spinning/rotation of particles requires unique properties of the illuminated particle, e.g., shape [23], birefringence [24], or specially structured illuminating beam, e.g., a circularly polarized beam [25,26] or a vortex beam [27,28]. The transverse spinning of metallic particles is intrinsically different from optical spinning utilizing the transfer of photon spin or orbital angular momentum [24,25,27,29], or based on the specially designed shape of the trapped particle [23], as it is induced by the vortex-like force field. Experimental results show a good agreement with the theoretical predictions: the gold microparticles were observed to be confined at two off-axis positions symmetric about the trap center and to spin transversely at a frequency of
Sign up for Photonics Research TOC. Get the latest issue of Photonics Research delivered right to you!Sign up now
2. METHODS AND MATERIALS
The experiment was conducted with an inverted optical tweezers setup as illustrated in Fig. 1(a). A collimated Gaussian beam polarized along the
Figure 1.Principle of the off-axis levitation and the transverse spinning of metallic microparticles. (a) Sketch for an inverted optical tweezers setup. (b) The principle of off-axis optical levitation of metallic microparticles. The scattering force along the
Figures 1(b)–1(d) present the principle of off-axis optical trapping and transverse spinning of metallic microparticles using a Gaussian trap. For large-size metallic particles, scattering force dominates and tends to push the particle out of the beam’s center, prohibiting on-axis trapping. However, a careful examination shows that off-axis confinement at the edge of the focal region is possible when considering a joint action of several mechanisms as shown in Fig. 1(b). At this position, the axial scattering force is balanced by the particle’s gravity (reduced by buoyancy), while the transverse scattering force is negligibly small so that the transverse gradient force can overcome the repelling effect. When departing this position, the particle will be pulled back by a restoring force [provided by a vortex-like force field shown in Figs. 2(b) and 2(c)] as discussed below. In such an asymmetric force field, the off-axis confined particle will spin about the transverse
Figure 2.Simulated force fields acting on a gold particle with a radius of 1.5 μm trapped in water with trapping power of 10 mW. (a) Spatial distribution of the magnitude of the 2D net force
In simulations, the time-averaged optical force
3. RESULTS AND DISCUSSION
A. Simulation Results
The total optical force on the particle was computed via the integral in Eq. (1), where the incident focused fields were calculated with Richards–Wolf integral [37,38], and the scattered fields were calculated based on Eqs. (3) and (4) by using the
To demonstrate the existence of off-axis equilibrium positions, we calculated the two-dimensional (2D) force distributions in the
Interestingly, however, there are two equilibrium positions (locations of zero net forces) at
To judge whether the above zero force positions, i.e.,
Figure 3.The net forces experienced by a gold particle with a radius
The off-axis levitated metallic particle tends to be more stable than the on-axis levitated one that can easily escape from the trap in the following sense. When the illumination power is changed, stable off-axis confinement still exists, although its position will change. For example, for the power
In addition to stable confinement, another appealing property of off-axis levitation is the vortex-like force field experienced by the particle as demonstrated in Fig. 2. When being confined at the stable equilibrium positions, the particle is expected to execute a transverse spinning motion. The calculated spin torque on the particle with
B. Experimental Results
1. Off-axis Levitation
The above numerical results predict that a gold microparticle will be stably confined at two edge positions of a focused Gaussian beam spot. Figure 4 presents the time-lapse images of two gold microparticles (
Figure 4.Experimental results of off-axis 3D optical confinement and manipulation of gold microparticles with the radius
Figure 5.Equilibrium positions of the off-axis confined gold microparticle for various laser power (see
As predicted by the above simulations, the off-axis levitated metallic particles are more stable than the on-axis levitated ones concerning changes in the trapping power. To validate this, we investigated the behavior of the off-axis confined particle under different laser power (Fig. 5). Figure 5(a) shows that the lateral displacement
The trend shown in Figs. 5(a) and 5(b) indicates that there is no high-power limit on the trapping stability. Therefore the off-axis levitated particle has higher trapping stability than the on-axis levitated one [20]. The low-power limit is given by the requirement that the scattering force is large enough to cancel the gravity of the particle and defeat the Brownian motion as well. The experimental results confirm the particle’s behavior under different illumination power [Fig. 5(c) and
Figure 6.Transverse spinning of gold microparticle assembly using a linearly polarized Gaussian beam (see
2. Off-Axis Transverse Spinning
Besides trapping, the spinning of particles also attracts wide attention because of its wide applications and the interesting physics behind it. Compared to longitudinal spinning about the beam propagation direction, which has been widely investigated [42,43], transverse spinning of the particle about the axis perpendicular to the beam propagation direction is still at its infancy and will gain continually growing attention in the future. Our theoretical analysis has suggested a transverse spinning of an off-axis trapped gold particle due to the asymmetric force field, unlike that utilizing transfer of photon spin or orbital angular momentum or the particle’s shape. Experimental results indicate transverse spinning of a single quasi-spherical gold microparticle by the periodic motion (
However, although we can distinguish the spinning motion, it is difficult to determine the spinning rate. To provide clear spinning results, we selected an asymmetric particle assembly as the candidate, formed by two large (
By using a linearly polarized Gaussian beam, metallic particles were demonstrated to be confined at the two equilibrium positions shown in Fig. 4. When the particles are placed at other positions near these two equilibrium positions, they tend to be attracted to the equilibrium positions [Fig. 2(i)]. This feature can be exploited to assemble metallic microparticles along arcs centered at the equilibrium positions (see
4. CONCLUSION
In summary, we have reported and characterized a new mechanism for the optical manipulation of metallic (gold) microparticles. Specifically, we demonstrated the off-axis optical levitation of metallic microparticles using a linearly polarized Gaussian beam by theoretical analysis (Figs. 2 and 3) and experimental measurements (Figs. 4 and 5). The off-axis levitation of metallic microparticles is much more stable than the on-axis levitation [18–20]. While being confined off the axis of the Gaussian beam, the metallic microparticle has been demonstrated to do transverse spinning simultaneously as a result of the asymmetric force field (Fig. 6). This transversely spinning metallic microparticle can serve as a new source of controllable micro-flow generation or a new kind of spanner.
Moreover, although the gold particle has a large imaginary component of the dielectric constant, there seems no significant influence of the heating of the particle on the stable confinement. In the experiments, we have not observed the escape of the particles from the trap when confined in the trap for a few minutes. The change of the stable equilibrium positions results in the magnitude of intensity always being low enough to introduce heating effects, such as convection and bubble formation. We believe that the off-axis confinement of metallic particles will serve as a promising tool for many applications, for example, to construct dynamically tunable or reconfigurable metamaterials in material science [45].
References
[1] E. A. Coronado, E. R. Encina, F. D. Stefani. Optical properties of metallic nanoparticles: manipulating light, heat and forces at the nanoscale. Nanoscale, 3, 4042-4059(2011).
[2] J. Meena, A. Saneja, A. Gupta, A. K. Panda, R. Ahuja, E. Lichtfouse, A. K. Panda, S. Bhaskar. Inorganic particles for delivering natural products. Sustainable Agriculture Reviews 44: Pharmaceutical Technology for Natural Products Delivery, 2, 205-241(2020).
[3] S. K. Dondapati, T. K. Sau, C. Hrelescu, T. A. Klar, F. D. Stefani, J. Feldmann. Label-free biosensing based on single gold nanostars as plasmonic transducers. ACS Nano, 4, 6318-6322(2010).
[4] P. Bharadwaj, B. Deutsch, L. Novotny. Optical antennas. Adv. Opt. Photon., 1, 438-483(2009).
[5] V. Kotaidis, A. Plech. Cavitation dynamics on the nanoscale. Appl. Phys. Lett., 87, 213102(2005).
[6] W. Huang, W. Qian, M. A. El-Sayed. Gold nanoparticles propulsion from surface fueled by absorption of femtosecond laser pulse at their surface plasmon resonance. J. Am. Chem. Soc., 128, 13330-13331(2006).
[7] D. Jaque, L. M. Maestro, B. Del Rosal, P. Haro-Gonzalez, A. Benayas, J. Plaza, E. M. Rodriguez, J. G. Sole. Nanoparticles for photothermal therapies. Nanoscale, 6, 9494-9530(2014).
[8] K. Svoboda, S. M. Block. Optical trapping of metallic Rayleigh particles. Opt. Lett., 19, 930-932(1994).
[9] D. Gao, W. Ding, M. Nieto-Vesperinas, X. Ding, M. Rahman, T. Zhang, C. Lim, C.-W. Qiu. Optical manipulation from the microscale to the nanoscale: fundamentals, advances and prospects. Light Sci. Appl., 6, e17039(2017).
[10] Q. Zhan. Trapping metallic Rayleigh particles with radial polarization. Opt. Express, 12, 3377-3382(2004).
[11] P. M. Hansen, V. K. Bhatia, N. Harrit, L. Oddershede. Expanding the optical trapping range of gold nanoparticles. Nano Lett., 5, 1937-1942(2005).
[12] J.-Q. Qin, X.-L. Wang, D. Jia, J. Chen, Y.-X. Fan, J. Ding, H.-T. Wang. FDTD approach to optical forces of tightly focused vector beams on metal particles. Opt. Express, 17, 8407-8416(2009).
[13] F. Hajizadeh, S. N. S. Reihani. Optimized optical trapping of gold nanoparticles. Opt. Express, 18, 551-559(2010).
[14] L. Huang, H. Guo, J. Li, L. Ling, B. Feng, Z.-Y. Li. Optical trapping of gold nanoparticles by cylindrical vector beam. Opt. Lett., 37, 1694-1696(2012).
[15] L. Jauffred, S. M. Taheri, R. Schmitt, H. Linke, L. B. Oddershede. Optical trapping of gold nanoparticles in air. Nano Lett., 15, 4713-4719(2015).
[16] H. Furukawa, I. Yamaguchi. Optical trapping of metallic particles by a fixed Gaussian beam. Opt. Lett., 23, 216-218(1998).
[17] P. C. Ke, M. Gu. Characterization of trapping force on metallic Mie particles. Appl. Opt., 38, 160-167(1999).
[18] M. Gu, D. Morrish, P. C. Ke. Enhancement of transverse trapping efficiency for a metallic particle using an obstructed laser beam. Appl. Phys. Lett., 77, 34-36(2000).
[19] M. Gu, D. Morrish. Three-dimensional trapping of Mie metallic particles by the use of obstructed laser beams. J. Appl. Phys., 91, 1606-1612(2002).
[20] Y. Zhang, X. Dou, Y. Dai, X. Wang, C. Min, X. Yuan. All-optical manipulation of micrometer-sized metallic particles. Photon. Res., 6, 66-71(2018).
[21] K. Sakai, S. Noda. Optical trapping of metal particles in doughnut-shaped beam emitted by photonic-crystal laser. Electron. Lett., 43, 107-108(2007).
[22] Z. Shen, L. Su, X. C. Yuan, Y. C. Shen. Trapping and rotating of a metallic particle trimer with optical vortex. Appl. Phys. Lett., 109, 241901(2016).
[23] P. Galajda, P. Ormos. Rotors produced and driven in laser tweezers with reversed direction of rotation. Appl. Phys. Lett., 80, 4653-4655(2002).
[24] R. L. Eriksen, P. J. Rodrigo, V. R. Daria, J. Glückstad. Spatial light modulator controlled alignment and spinning of birefringent particles optically trapped in an array. Appl. Opt., 42, 5107-5111(2003).
[25] P. L. Marston, J. H. Crichton. Radiation torque on a sphere caused by a circularly-polarized electromagnetic wave. Phys. Rev. A, 30, 2508-2516(1984).
[26] Y. Zhao, D. Shapiro, D. McGloin, D. T. Chiu, S. Marchesini. Direct observation of the transfer of orbital angular momentum to metal particles from a focused circularly polarized Gaussian beam. Opt. Express, 17, 23316-23322(2009).
[27] L. Allen, M. W. Beijersbergen, R. J. C. Spreeuw, J. P. Woerdman. Orbital angular momentum of light and the transformation of Laguerre-Gaussian laser modes. Phys. Rev. A, 45, 8185-8189(1992).
[28] A. T. O’Neil, M. J. Padgett. Three-dimensional optical confinement of micron-sized metal particles and the decoupling of the spin and orbital angular momentum within an optical spanner. Opt. Commun., 185, 139-143(2000).
[29] N. B. Simpson, K. Dholakia, L. Allen, M. J. Padgett. Mechanical equivalence of spin and orbital angular momentum of light: an optical spanner. Opt. Lett., 22, 52-54(1997).
[30] M. Padgett, R. Bowman. Tweezers with a twist. Nat. Photonics, 5, 343-348(2011).
[31] P. H. Jones, O. M. Maragò, G. Volpe. Optical tweezers: Principles and Applications(2015).
[32] P. C. Waterman. New formulation of acoustic scattering. J. Acoust. Soc. Am., 45, 1417-1429(1969).
[33] P. C. Waterman. Symmetry, unitarity, and geometry in electromagnetic scattering. Phys. Rev. D, 3, 825-839(1971).
[34] I. M. Michael, D. Larry. Scattering, Absorption and Emission of Light by Small Particles(2002).
[35] S. Yan, B. Yao. Transverse trapping forces of focused Gaussian beam on ellipsoidal particles. J. Opt. Soc. Am. B, 24, 1596-1602(2007).
[36] S. Yan, B. Yao. Radiation forces of a highly focused radially polarized beam on spherical particles. Phys. Rev. A, 76, 053836(2007).
[37] E. Wolf. Electromagnetic diffraction in optical systems. I. An integral representation of the image field. Proc. R. Soc. London A, 253, 349-357(1959).
[38] B. Richards, E. Wolf. Electromagnetic diffraction in optical systems. II. Structure of the image field in an aplanatic system. Proc. R. Soc. London A, 253, 358-379(1959).
[39] T. A. Nieminen, H. Rubinsztein-Dunlop, N. R. Heckenberg, A. I. Bishop. Numerical modelling of optical trapping. Comput. Phys. Commun., 142, 468-471(2001).
[40] S. Adachi. The Handbook on Optical Constants of Metals: In Tables and Figures(2012).
[41] M. Li, S. Yan, B. Yao, Y. Liang, G. Han, P. Zhang. Optical trapping force and torque on spheroidal Rayleigh particles with arbitrary spatial orientations. J. Opt. Soc. Am. A, 33, 1341-1347(2016).
[42] R. Reimann, M. Doderer, E. Hebestreit, R. Diehl, M. Frimmer, D. Windey, F. Tebbenjohanns, L. Novotny. GHz rotation of an optically trapped nanoparticle in vacuum. Phys. Rev. Lett., 121, 033602(2018).
[43] J. Ahn, Z. Xu, J. Bang, Y.-H. Deng, T. M. Hoang, Q. Han, R.-M. Ma, T. Li. Optically levitated nanodumbbell torsion balance and GHz nanomechanical rotor. Phys. Rev. Lett., 121, 033603(2018).
[44] G. Pesce, G. Volpe, A. C. D. Luca, G. Rusciano, G. Volpe. Quantitative assessment of non-conservative radiation forces in an optical trap. Europhys. Lett., 86, 38002(2009).
[45] H. Gao, Y. Xu, K. Yao, Y. Liu. Self-assembly of silica–gold core–shell microparticles by electric fields toward dynamically tunable metamaterials. ACS Appl. Mater. Interfaces, 13, 14417-14422(2021).
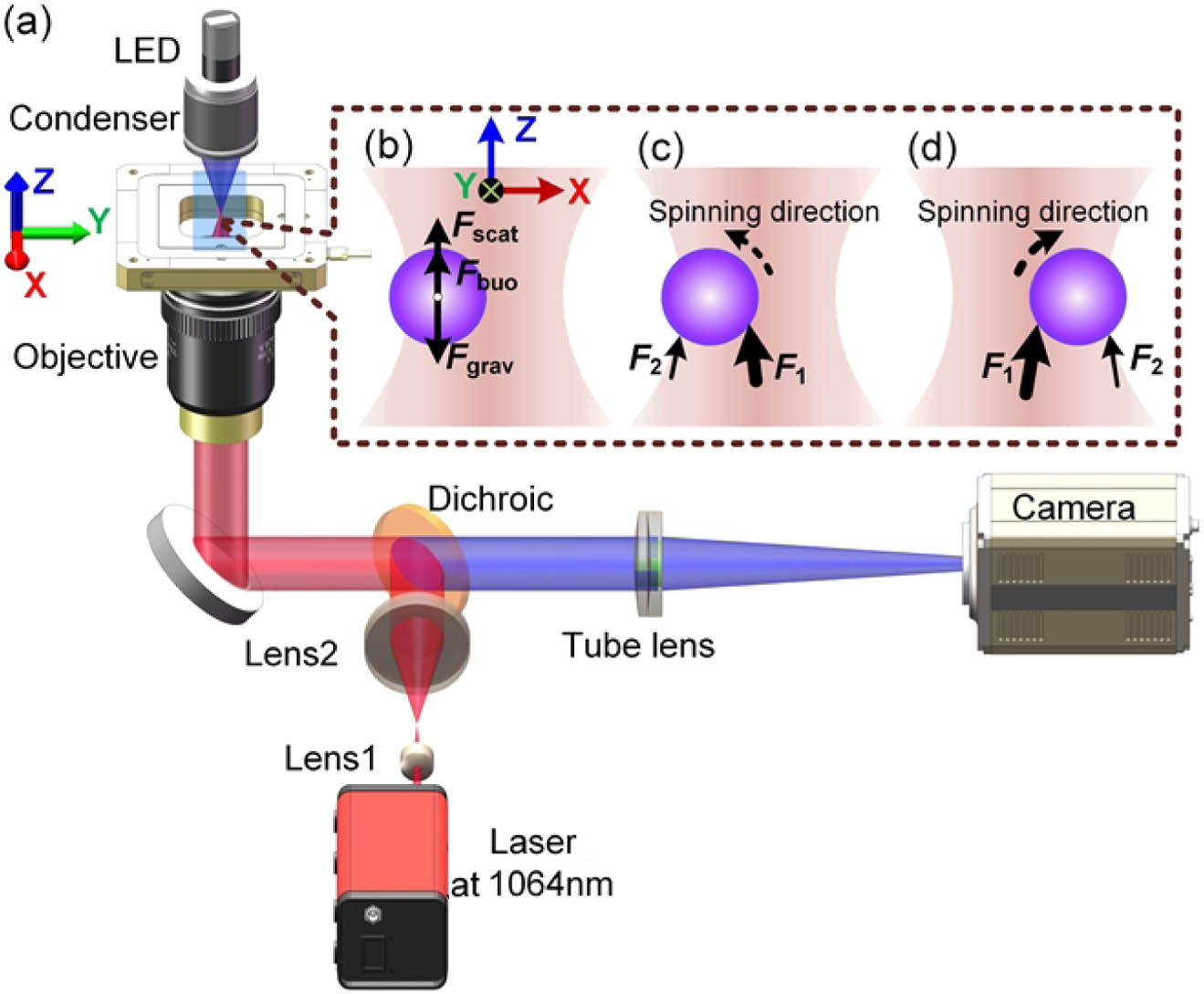
Set citation alerts for the article
Please enter your email address