Heming Wei, Zhangli Wu, Kexuan Sun, Haiyan Zhang, Chen Wang, Kemin Wang, Tian Yang, Fufei Pang, Xiaobei Zhang, Tingyun Wang, Sridhar Krishnaswamy, "Two-photon 3D printed spring-based Fabry–Pérot cavity resonator for acoustic wave detection and imaging," Photonics Res. 11, 780 (2023)

Search by keywords or author
- Photonics Research
- Vol. 11, Issue 5, 780 (2023)
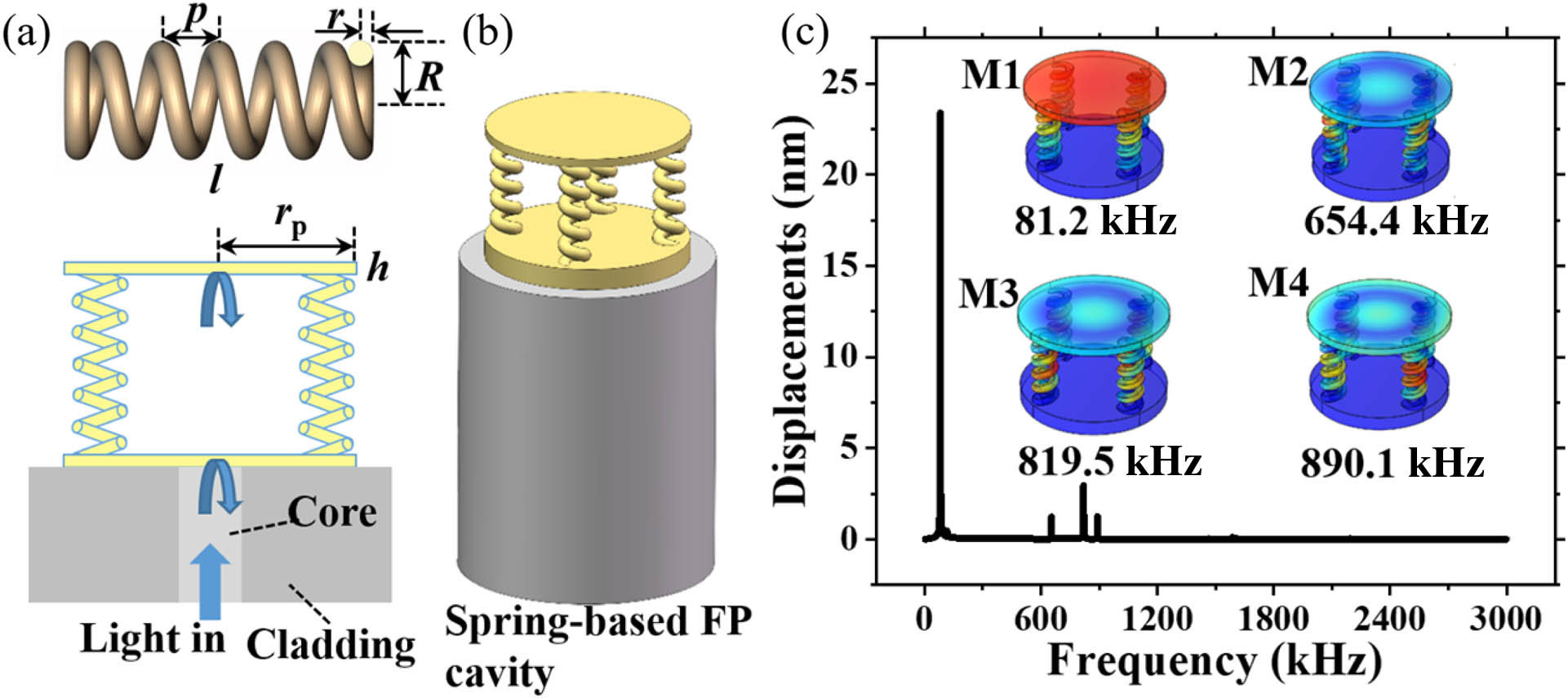
Fig. 1. Proposed spring-based FP optomechanical cavity resonator. (a) Schematic diagram of the spring resonator and the fiber-tip-based FP cavity where the springs are used as the supports with low stiffness. (b) Schematic diagram of the proposed FP cavity microresonator for acoustic wave detection and imaging. (c) Mode simulation of the proposed device used for acoustic wave sensing in a water environment. Four vibration modes are found within the range from 0 to 3 MHz when the parameters are initially set as r = 3 μm , R = 9 μm , p = 10 μm , N = 5 , r p = 50 μm , h = 5 μm : Mode 1 at 81.2 kHz (M1), Mode 2 at 654.4 kHz (M2), Mode 3 at 819.5 kHz (M3), and Mode 4 at 890.1 kHz (M4).
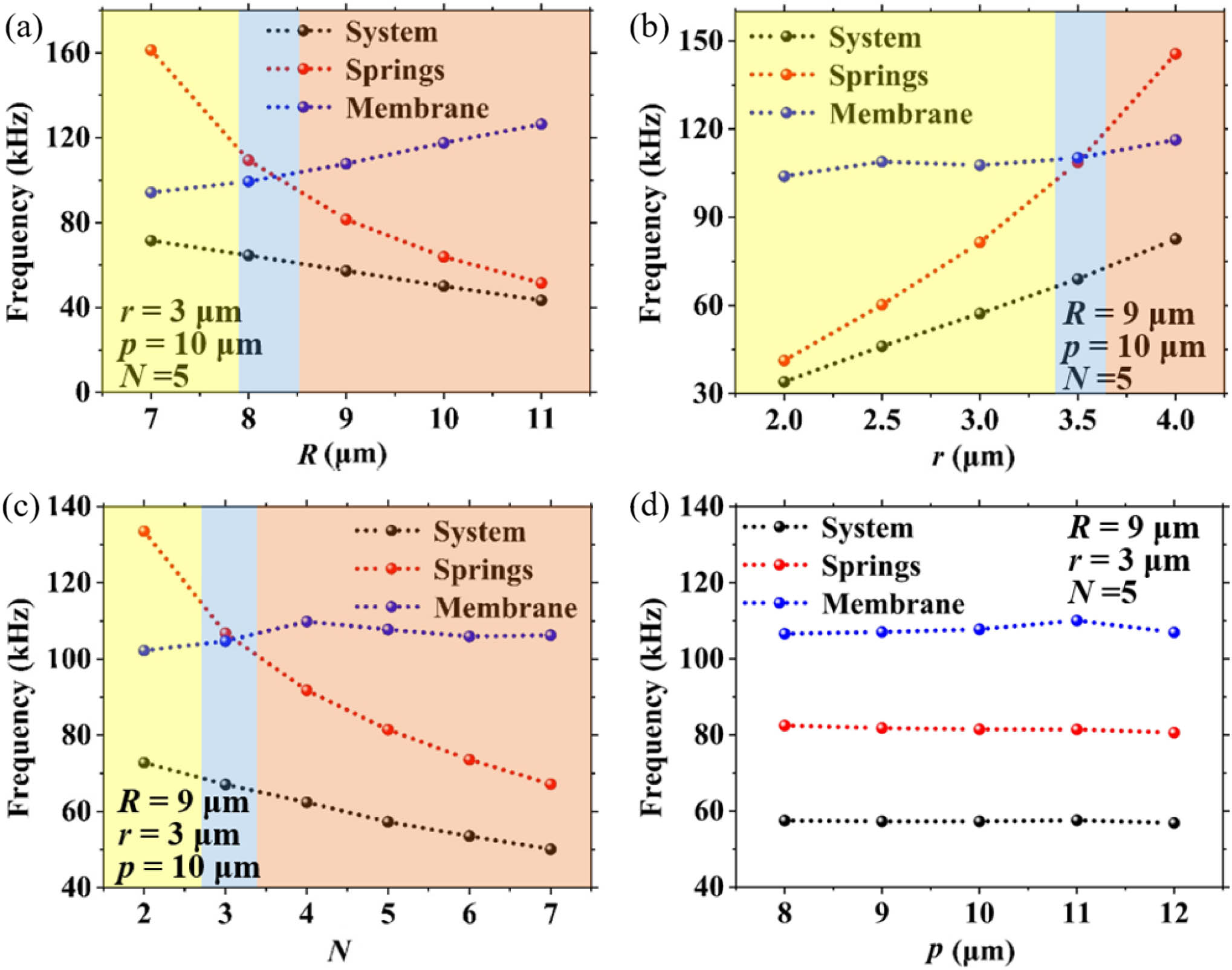
Fig. 2. Resonant frequency behavior of the whole device and its individual parts related to the parameters of (a) the outer radius, (b) the wire radius, (c) the coil number, and (d) the pitch. In these simulations, the resonant frequency of an individual part is studied assuming that the other part is rigidly constrained.
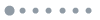
Fig. 3. Resonant frequency behavior of the device system and each part versus the plate membrane related to the parameters of the thickness within a range from (a) 0.5 to 10 μm and (b) 0.5 to 3 μm, where the outer radius is 9 μm and 7 μm.
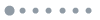
Fig. 4. Results of the 3D-printed devices. (a) Scanning electron images and (b) microscopic images of the fabricated microresonators. (c) and (d) Reflection spectra of the devices in atmospheric and aquatic environments.
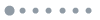
Fig. 5. Reflection spectra of the devices in atmospheric and aquatic environments. (a)–(c) Devices with a diaphragm thickness of 5 μm and an outer radius of (a) 7 μm, (b) 9 μm, and (c) 11 μm. (d)–(f) Devices with a diaphragm thickness of 1 μm and an outer radius of (d) 7 μm, (e) 9 μm, and (f) 11 μm.
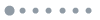
Fig. 6. Schematic diagram of the experimental setup for acoustic wave detection.
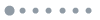
Fig. 7. Low frequency response of the device: (a) time response and (b) frequency response. (c) Acoustic response of the device to the sinusoidal burst signal with an amplitude of 82.5 Pa and a frequency of 75 kHz. The inset shows the detected time response.
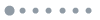
Fig. 8. (a) High acoustic response of the device to the sinusoidal burst signal. The inset shows a detected time signal at 750 kHz. (b) Acoustic wave pressure sensitivity and (c) angle response of the device.
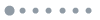
Fig. 9. (a) Schematic of the experimental setup. (b) Total focusing algorithm. (c) Detected signals at different detecting positions. (d) Reconstructed cross-section image of the objects.
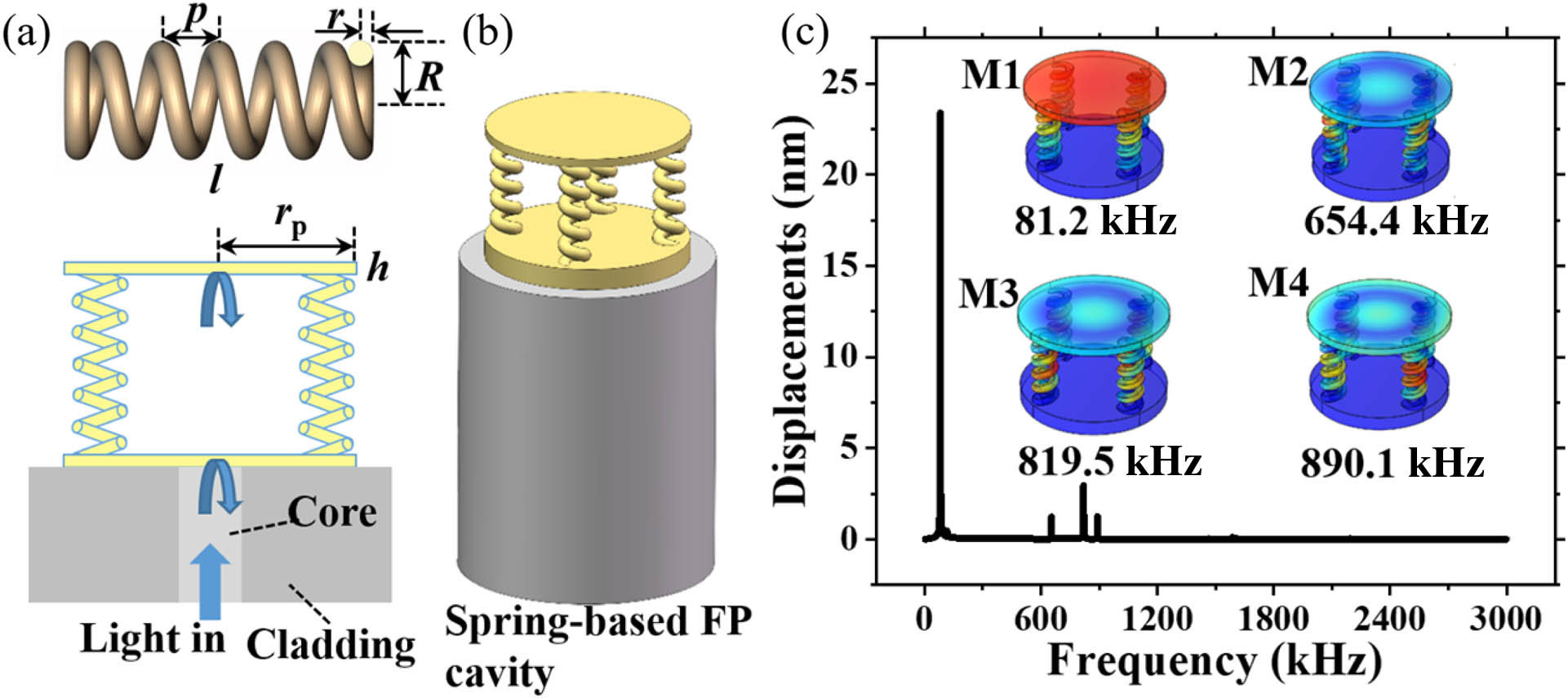
Set citation alerts for the article
Please enter your email address