
- Chinese Optics Letters
- Vol. 20, Issue 1, 013701 (2022)
Abstract
Keywords
1. Introduction
The electromagnetically induced transparency (EIT) effect, causing the appearance of a sharp transparent peak in the broad absorption or reflection spectrum, is attracting considerable research attention due to the self-enhanced nonlinearity, refractive index, and ultraslow group velocity. However, the application of the EIT effect is constrained by the extreme quantum condition. The analogue of EIT effect may be applied to other optical scales, such as the terahertz (THz) regime[
More recent attention has focused on the multi-band PIT effect in THz metamaterials, with multiple transparent windows induced by the coupling effect between THz resonators[
In this work, we numerically and experimentally study an ultrafast all-optical switching of the PIT effect in a metaphotonic device. A CW resonator and two pairs of SRRs are implemented in such a device to support the bright and dark modes that can destructively interfere with each other, resulting in the generation of the PIT phenomenon. In order to switch the individual PIT effect, we incorporate the long lateral metal bars (LLMBs) and silicon (Si) islands into the CW resonator and SRRs. Additionally, taking advantage of the broken
Sign up for Chinese Optics Letters TOC. Get the latest issue of Chinese Optics Letters delivered right to you!Sign up now
2. Experiments
2.1. Sample characterization
The fabrication of a metamaterial device is processed as follows. A standard Si-on-sapphire (SOS) wafer was selected as the substrate. The SOS wafer consists of sapphire layer and Si layer with thicknesses of 525 µm and 500 nm, respectively. A photoresist layer was spun on the cleaned SOS wafer and drawn by a laser direct writing setup. After that, the photoresist layer was processed by soft bake. Then, the metal chromium (Cr) and gold (Au) were sputtered onto the SOS wafer in order as a coherent layer and metamaterial, respectively. The total height of the metal structure was about 150 nm. After processing by the lift-off technique, the photoresist layer resolved and left an intact metamaterial device. The epitaxial Si was processed by dry etching.
2.2. Numerical simulation
The simulation of the THz probe on the metamaterial device is performed using the classical finite element method. The THz probe is set, transmitting normally to the surface of the metamaterial device, the electric-field intensity of which is set as 1 V/m. The permittivity of sapphire is set as 10.5, from the comparison of THz pulse delay between the absence and presence of a sapphire in the passing way. The permittivity of Si is set as 11.7. In addition, the conductivity of Au is set as
2.3. Measurements in OPTP system
As an active control sample of the THz metamaterial, we characterize the dynamic response using a commercial OPTP time-domain spectral system (TTT-02-OPTP, from TuoTuo Technology). After the Ti:sapphire amplifier, the output beam produces femtosecond pulses with a width of
Figure 1.(a) Schematic illustration of the metaphotonic device with triatomic structural molecules for OPTP spectroscopy. The metadevice is probed by THz beams at normal incidence and excited by an optical pump at oblique incidence. (b) Unit cell of the metamaterial comprising a CW resonator, an LLMB, and two pairs of SRRs. The geometrical parameters are as follows: Px = Py = 100, w = 5, g = 4, q = 12, L1 = 80, L2 = 38, L3 = 20, and L4 = 17 µm. (c) Optical microscopic image of the metamaterials.
3. Results and Discussion
Figure 2(a) shows the temporal evolution of the THz transmission spectra of the metadevice, at an optical pump power of
Figure 2.(a) Experimentally measured THz transmission spectra of the metadevice at various pump-probe delays (as labeled). (b) Color map of THz transient transmissions against pump-probe delay and frequency.
We proceed to explore the impact of pump power on the modulation of the PIT effect. Figure 3 illustrates the vivid comparison between the numerically calculated transmission spectra and the experimentally measured results at a pump-probe delay time of 6 ps. The blue curve in Fig. 3(a) represents a typical PIT effect that is characterized by a transparent window under a broad absorption background in the absence of a laser pump. With the increment of optical pump power, the PIT effect is gradually suppressed, since a large amount of free carriers are photoexcited into the conduction band, activating the quasi-dark resonance mode and shielding the destructive coupling between the dark resonance mode and bright resonance mode. At a pump power of
Figure 3.(a)–(d) Spectral dispersion of measured THz transmission for the triatomic metamaterials with a series of selected pump power levels (as labeled) at a fixed pump-probe delay of 6 ps for which the photo-induced change in spectral dispersion is maximized. (e)–(h) Corresponding numerically simulated transmission spectra varying with the conductivity of Si (as labeled).
Due to the tunable dip in the PIT window, a remarkable variation of slow light effect could be controlled in our designed metadevice. The slow light effect is usually represented by a group delay,
Figure 4.(a)–(e) Numerically calculated and (f)–(j) experimentally measured group delays of the THz waves transmitted through the metadevice for a series of conductivity of Si and pump power (as labeled), respectively.
To understand the evolution of the PIT effect in the triatomic metamaterial, we calculate the transmission response and electric-field profiles in CW resonators and triatomic metamaterials, with the results presented by Fig. 5. Figure 5(a) signifies the simulated transmission response of a single CW resonator. The localized surface plasmon (LSP) resonance is excited in CW resonators and leads to a transparent dip at 0.72 THz. By placing the SRR and LLMB around the CW resonator and across the CW resonator, respectively, we realize the PIT effect with an apparent transparent peak at 0.71 THz. The near-field coupling between CW resonators and SRRs excites the SRRs on both sides of CW resonators. As a result, the PIT effect comes from the destructive interference between the LSP resonance and the inductive-capacitive (LC) resonance, which are supported by the CW resonator and SRRs, respectively. Here, the CW resonators and SRRs act as the bright mode and dark mode, respectively. Figures 5(b) and 5(e) show the transmission spectrum and electric-field profiles. In order to accomplish a three-level coupling interaction in the triatomic metamaterials, we introduce the Si islands on a couple of splits in SRRs [shown in Fig. 1(b)]. By injecting laser pumps onto the Si islands, a couple of SRRs are short-circuited by photo-induced carriers in Si. Therefore, the destructive interference between CW resonators and half of the SRRs is suppressed. For lack of local coupling between electric fields in CW resonators and short-circuited SRRs, another type of LSP resonance is generated between the LLMB and CW resonator, because of the asymmetry in the triatomic metamaterials. By simulating the distributions of surface current density at the peak of individual PIT effects and the dip of dual-band PIT effects, respectively, as shown in Fig. 6, we can intuitively understand the underlying physics in switching of the PIT effect. For the case without pump, the dark mode that is coupled with the bright mode occupies the main resonant mode [see Fig. 6(a)]. Nevertheless, when under optical pump irradiation, the quasi-dark mode is excited, whereas the surface current mainly focuses on the LLMB and CW resonator [see Fig. 6(b)]. Accompanied by the new LSP resonance, a transparent dip occurs at the original transparent peak, switching the PIT effect from individual window into dual windows, as exhibited in Fig. 5(c).
Figure 5.Simulated THz transmission spectra through (a) CW resonators, triatomic metamaterials (b) in the dark and (c) pumped by laser pulses. The conductivity of Si is selected as 10 S/m (in the dark) and 80,000 S/m (under laser pump). (d)–(f) Corresponding electric-field profiles in the triatomic metamaterial.
Figure 6.Distribution of surface current density of metamaterials at resonance frequency. (a) Surface current at the transparent peak for σSi = 10 S/m (case without pump). (b) Surface current at the transparent dip for σSi = 80,000 S/m (case with the highest pump fluence).
4. Conclusion
In summary, we have proposed a triatomic metamaterial device comprising CW resonators, SRRs, and LLMBs and hybridized with Si islands. An individual PIT effect occurs when THz waves transmit through the metaphotonic device, owing to the coupling effect between the bright mode and dark mode supported by CW resonators and SRRs. Excited by optical pumps, a pair of SRRs are short-circuited by photo-induced carriers in Si islands, promoting the occurrence of the quasi-dark mode supported by CW resonator and LLMB. Simultaneously, the switching of the PIT effect from individual transparent windows to dual-band windows is also achieved. A prominent slow light effect is measured during the modulating process. Benefiting from the ultrafast relaxation time of carriers in epitaxial Si, the recovery time of the modulating process is in a nanosecond timescale.
References
[1] T.-T. Kim, H.-D. Kim, R. Zhao, S. S. Oh, T. Ha, D. S. Chung, Y. H. Lee, B. Min, S. Zhang. Electrically tunable slow light using graphene metamaterials. ACS Photon., 5, 1800(2018).
[2] H. Jung, H. Jo, W. Lee, B. Kim, H. Choi, M. S. Kang, H. Lee. Electrical control of electromagnetically induced transparency by terahertz metamaterial funneling. Adv. Opt. Mater., 7, 1801205(2019).
[3] K. M. Devi, M. Islam, D. R. Chowdhury, A. K. Sarma, G. Kumar. Plasmon-induced transparency in graphene-based terahertz metamaterials. EPL, 120, 27005(2017).
[4] J. Gu, R. Singh, X. Liu, X. Zhang, Y. Ma, S. Zhang, S. A. Maier, Z. Tian, A. K. Azad, H.-T. Chen, A. J. Taylor, J. Han, W. Zhang. Active control of electromagnetically induced transparency analogue in terahertz metamaterials. Nat. Commun., 3, 1151(2012).
[5] A. Ahmadivand, B. Gerislioglu, Z. Ramezani. Gated graphene island-enabled tunable charge transfer plasmon terahertz metamodulator. Nanoscale, 11, 8091(2019).
[6] M. Manjappa, Y. K. Srivastava, A. Solanki, A. Kumar, T. C. Sum, R. Singh. Hybrid lead halide perovskites for ultrasensitive photoactive switching in terahertz metamaterial devices. Adv. Mater., 29, 1605881(2017).
[7] P. Pitchappa, A. Kumar, S. Prakash, H. Jani, T. Venkatesan, R. Singh. Chalcogenide phase change material for active terahertz photonics. Adv. Mater., 31, 1808157(2019).
[8] H.-T. Chen, J. F. O’Hara, A. K. Azad, A. J. Taylor, R. D. Averitt, D. B. Shrekenhamer, W. J. Padilla. Experimental demonstration of frequency-agile terahertz metamaterials. Nat. Photon., 2, 295(2008).
[9] N.-H. Shen, M. Massaouti, M. Gokkavas, J.-M. Manceau, E. Ozbay, M. Kafesaki, T. Koschny, S. Tzortzakis, C. M. Soukoulis. Optically implemented broadband blueshift switch in the terahertz regime. Phys. Rev. Lett., 106, 037403(2011).
[10] Z. Chen, X. Chen, L. Tao, K. Chen, M. Long, X. Liu, K. Yan, R. I. Stantchev, E. Pickwell-MacPherson, J.-B. Xu. Graphene controlled Brewster angle device for ultra broadband terahertz modulation. Nat. Commun., 9, 4909(2018).
[11] M. D. Goldflam, M. K. Liu, B. C. Chapler, H. T. Stinson, A. J. Sternbach, A. S. McLeod, J. D. Zhang, K. Geng, M. Royal, B.-J. Kim, R. D. Averitt, N. M. Jokerst, D. R. Smith, H.-T. Kim, D. N. Basov. Voltage switching of a VO2 memory metasurface using ionic gel. Appl. Phys. Lett., 105, 041117(2014).
[12] H. Jung, J. Koo, E. Heo, B. Cho, C. In, W. Lee, H. Jo, J. H. Cho, H. Choi, M. S. Kang, H. Lee. Electrically controllable molecularization of terahertz meta-atoms. Adv. Mater., 30, 1802760(2018).
[13] Y. Hu, T. Jiang, J. Zhou, H. Hao, H. Sun, H. Ouyang, M. Tong, Y. Tang, H. Li, J. You, X. Zheng, Z. Xu, X. Cheng. Ultrafast terahertz transmission/group delay switching in photoactive WSe2-functionalized metaphotonic devices. Nano Energy, 68, 104280(2020).
[14] J. Zhou, Y. Hu, T. Jiang, H. Ouyang, H. Li, Y. Sui, H. Hao, J. You, X. Zheng, Z. Xu, X. Cheng. Ultrasensitive polarization-dependent terahertz modulation in hybrid perovskites plasmon-induced transparency devices. Photon. Res., 7, 994(2019).
[15] J. Ji, S. Zhou, W. Wang, F. Ling, J. Yao. Active control of terahertz plasmon-induced transparency in the hybrid metamaterial/monolayer MoS2/Si structure. Nanoscale, 11, 9429(2019).
[16] P. Pitchappa, M. Manjappa, C. P. Ho, R. Singh, N. Singh, C. Lee. Active control of electromagnetically induced transparency analog in terahertz MEMS metamaterial. Adv. Opt. Mater., 4, 541(2016).
[17] C. Liu, P. Liu, C. Yang, Y. Lin, H. Liu. Analogue of dual-controlled electromagnetically induced transparency based on a graphene metamaterial. Carbon, 142, 354(2019).
[18] S. J. Kindness, N. W. Almond, B. Wei, R. Wallis, W. Michailow, V. S. Kamboj, P. Braeuninger-Weimer, S. Hofmann, H. E. Beere, D. A. Ritchie, R. Degl’Innocenti. Active control of electromagnetically induced transparency in a terahertz metamaterial array with graphene for continuous resonance frequency tuning. Adv. Opt. Mater., 6, 1800570(2018).
[19] Y. Wu, C. La-o-vorakiat, X. Qiu, J. Liu, P. Deorani, K. Banerjee, J. Son, Y. Chen, E. E. M. Chia, H. Yang. Graphene terahertz modulators by ionic liquid gating. Adv. Mater., 27, 1874(2015).
[20] W. Y. Kim, H.-D. Kim, T.-T. Kim, H.-S. Park, K. Lee, H. J. Choi, S. H. Lee, J. Son, N. Park, B. Min. Graphene–ferroelectric metadevices for nonvolatile memory and reconfigurable logic-gate operations. Nat. Commun., 7, 10429(2016).
[21] Z. Xu, Y.-S. Lin. A stretchable terahertz parabolic‐shaped metamaterial. Adv. Opt. Mater., 7, 1900379(2019).
[22] J. Ji, S. Zhou, W. Wang, C. Luo, Y. Liu, F. Ling, J. Yao. Active multifunctional terahertz modulator based on plasmonic metasurface. Opt. Express, 27, 2363(2019).
[23] S. H. Lee, M. Choi, T.-T. Kim, S. Lee, M. Liu, X. Yin, H. K. Choi, S. S. Lee, C.-G. Choi, S.-Y. Choi, X. Zhang, B. Min. Switching terahertz waves with gate-controlled active graphene metamaterials. Nat. Mater., 11, 936(2012).
[24] M. M. Jadidi, A. B. Sushkov, R. L. Myers-Ward, A. K. Boyd, K. M. Daniels, D. K. Gaskill, M. S. Fuhrer, H. D. Drew, T. E. Murphy. Tunable terahertz hybrid metal–graphene plasmons. Nano Lett., 15, 7099(2015).
[25] S. Xiao, T. Wang, T. Liu, C. Zhou, X. Jiang, J. Zhang. Active metamaterials and metadevices: a review. J. Phys. D: Appl. Phys., 53, 503002(2020).
[26] P. Tassin, L. Zhang, T. Koschny, E. N. Economou, C. M. Soukoulis. Low-loss metamaterials based on classical electromagnetically induced transparency. Phys. Rev. Lett., 102, 053901(2009).
[27] Q. Li, Z. Tian, X. Zhang, N. Xu, R. Singh, J. Gu, P. Lv, L.-B. Luo, S. Zhang, J. Han, W. Zhang. Dual control of active graphene-silicon hybrid metamaterial devices. Carbon, 90, 146(2015).
[28] S. Zhang, D. A. Genov, Y. Wang, M. Liu, X. Zhang. Plasmon-induced transparency in metamaterials. Phys. Rev. Lett., 101, 047401(2008).
[29] H. Sun, Y. Hu, Y. Tang, J. You, J. Zhou, H. Liu, X. Zheng. Ultrafast polarization-dependent all-optical switching of germanium-based metaphotonic devices. Photon. Res., 8, 263(2020).
[30] H. Sun, J. Yang, H. Liu, D. Wu, X. Zheng. Process-controllable modulation of plasmon-induced transparency in terahertz metamaterials. Chin. Opt. Lett., 19, 013602(2021).
[31] H. Sun, Y. Tang, Y. Hu, J. You, H. Liu, X. Zheng. Active formatting modulation of electromagnetically induced transparency in metamaterials. Chin. Opt. Lett., 18, 092402(2020).
[32] Y. Hu, T. Jiang, J. Zhou, H. Hao, H. Sun, H. Ouyang, M. Tong, Y. Tang, H. Li, J. You, X. Zheng, Z. Xu, X. Cheng. Ultrafast terahertz frequency and phase tuning by all‐optical molecularization of metasurfaces. Adv. Opt. Mater., 7, 1901050(2019).
[33] J. Zhou, C. Zhang, Q. Liu, J. You, X. Zheng, X. Cheng, T. Jiang. Controllable all-optical modulation speed in hybrid silicon-germanium devices utilizing the electromagnetically induced transparency effect. Nanophotonics, 9, 2797(2020).
[34] Y. Hu, T. Jiang, H. Sun, M. Tong, J. You, X. Zheng, Z. Xu, X. Cheng. Ultrafast frequency shift of electromagnetically induced transparency in terahertz metaphotonic devices. Laser Photon. Rev., 14, 1900338(2020).
[35] Y. Hu, J. You, M. Tong, X. Zheng, Z. Xu, X. Cheng, T. Jiang. Pump‐color selective control of ultrafast all‐optical switching dynamics in metaphotonic devices. Adv. Sci., 7, 2000799(2020).
[36] R. Sarkar, D. Ghindani, K. M. Devi, S. S. Prabhu, A. Ahmad, G. Kumar. Independently tunable electromagnetically induced transparency effect and dispersion in a multi-band terahertz metamaterial. Sci. Rep., 9, 18068(2019).
[37] J. Ding, B. Arigong, H. Ren, M. Zhou, J. Shao, M. Lu, Y. Chai, Y. Lin, H. Zhang. Tuneable complementary metamaterial structures based on graphene for single and multiple transparency windows. Sci. Rep., 4, 6128(2014).
[38] Y. Wang, M. Tao, Z. Pei, X. Yu, B. Wang, J. Jiang, X. He. Tunable bandwidth of double electromagnetic induced transparency windows in terahertz graphene metamaterial. RSC Adv., 8, 37057(2018).
[39] K. Zhang, C. Wang, L. Qin, R.-W. Peng, D.-H. Xu, X. Xiong, M. Wang. Dual-mode electromagnetically induced transparency and slow light in a terahertz metamaterial. Opt. Lett., 39, 3539(2014).
[40] F. Bagci, B. Akaoglu. Single and multi-band electromagnetically induced transparency-like effects with a four-fold symmetric metamaterial design. Mater. Res. Express, 6, 055806(2019).
[41] K. M. Devi, D. R. Chowdhury, G. Kumar, A. K. Sarma. Dual-band electromagnetically induced transparency effect in a concentrically coupled asymmetric terahertz metamaterial. J. Appl. Phys., 124, 063106(2018).
[42] C. Sun, J. Si, Z. Dong, X. Deng. Tunable multispectral plasmon induced transparency based on graphene metamaterials. Opt. Express, 24, 11466(2016).
[43] S. Hu, H. Yang, S. Han, X. Huang, B. Xiao. Tailoring dual-band electromagnetically induced transparency in planar metamaterials. J. Appl. Phys., 117, 043107(2015).
[44] C. Tang, Q. Niu, B.-X. Wang, W.-Q. Huang. Design of dual-band plasmon-induced transparent effect based on composite structure of closed-ring and square patch. Plasmonics, 14, 533(2019).
[45] Z. Dong, C. Sun, J. Si, X. Deng. Tunable polarization-independent plasmonically induced transparency based on metal-graphene metasurface. Opt. Express, 25, 12251(2017).
[46] J. Kim, R. Soref, W. R. Buchwald. Multi-peak electromagnetically induced transparency (EIT)-like transmission from bull’s-eye-shaped metamaterial. Opt. Express, 18, 17997(2010).
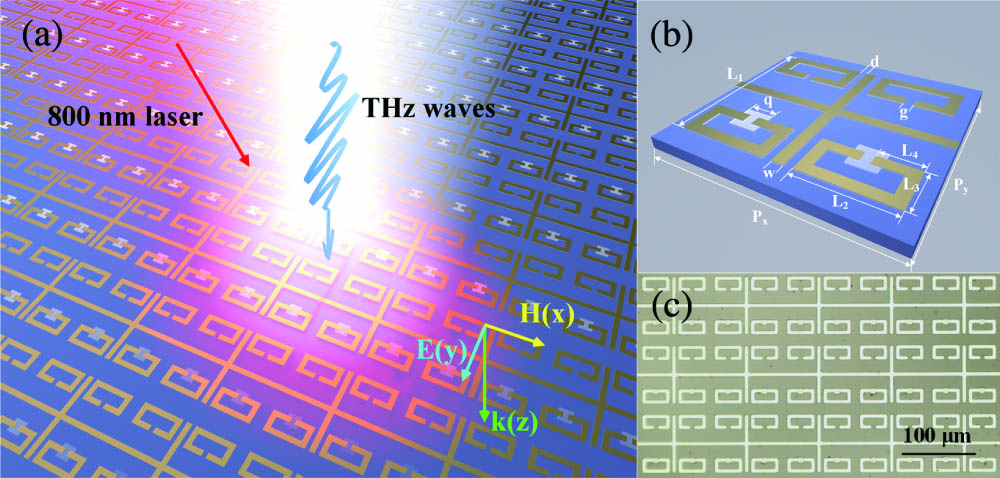
Set citation alerts for the article
Please enter your email address