Abstract
We present an ultrabroadband, high-speed wavelength-swept source based on a self-modulated femtosecond oscillator. Photonic crystal fiber was pumped by a mode-locked laser, resulting in a strong spectral broadening from 485 to 1800 nm. The pump laser cavity could be realigned in order to achieve total mode-locking of the longitudinal and transverse and electromagnetic modes. This led to spatial oscillations of the output beam, which induced modulation of the coupling efficiency to the fiber. Due to the fact that nonlinear spectral broadening was intensity dependent, this mechanism introduced wavelength sweeping at the fiber output. The sweeping rate could be adjusted between 7 and 21.5 MHz by changing the geometry of the pump cavity. By controlling the ratio of the transverse mode amplitudes, we were able to tune the sweeping bandwidth, eventually covering both the 1300 nm and 1700 nm bioimaging transparency windows. When compared with previously demonstrated wavelength-swept sources, our concept offers much broader tunability and higher speed. Moreover, it does not require an additional intensity modulator.1. INTRODUCTION
Wavelength-swept laser sources have numerous applications in biomedical sciences, e.g., frequency-domain fluorescence microscopy [1], swept-source phase microscopy [2], and optical coherence tomography (OCT) [3,4]. Among these bioimaging methods, the latter is definitely the most frequently employed in real-life clinical practice, and it typically makes it possible to obtain micrometer (μm) resolution with a tissue penetration depth of several millimeters (mm). Typically, OCT systems operate at three different IR transmission windows: [5], [4], and [6]. The long-wavelength range exhibits the highest water absorption; however, it has been demonstrated that due to the reduced impact of scattering it can support superior penetration depth not only in low-water-level samples [6–8]. Except for the spectral emission, which should be appropriate for the specific transmission window, the other crucial parameters of the employed swept source are the wavelength tunability range (which defines the achievable resolution) and the sweeping rate (determining the image acquisition time). Explicitly, it is favorable to use a broadband source with as high a tuning speed as possible.
Several various wavelength-swept sources have already been demonstrated. One approach relies on a laser employing a mechanically tunable optical filter [9]; however, these devices suffer from relatively low tuning speed, which is limited to several tens of kilohertz (kHz). Higher sweeping rates up to 1 MHz can be achieved with actively mode-locked lasers relying on dispersion tuning [10,11] and Fourier-domain mode-locking [12]. An entirely different approach is based on soliton self-frequency shift (SSFS) of ultrashort pulses in a highly nonlinear photonic crystal fiber (PCF). When a femtosecond high-intensity pulse propagates along the fiber, the process of intrapulse stimulated Raman scattering induces soliton spectral redshift. Importantly, the shift is proportional to the intensity of the coupled radiation, and thus the central wavelength of the emitted beam can be tuned simply by controlling the input power [13]. The magnitude of the shift can amount to a few hundred nanometers, while the pulse maintains its shape as well as coherence [14–17]. Simultaneously, the blueshifted part of the spectrum can be also broadened due to dispersive wave (Cherenkov radiation) generation [18,19]. Raman SSFS has been exploited for wavelength-swept infrared laser sources: the output of a mode-locked laser is coupled to PCF, and the input power is controlled with an acousto-optical modulator [20,21]. With an ytterbium-based pumping, tunability of 220 nm at 1220 nm has been achieved with modulation frequency of 1.3 MHz, and it has been subsequently applied for OCT measurements [22,23]. A time-averaged spectrum measurement of the output from such laser sources usually exhibits a smooth supercontinuum-like shape. Due to the lack of temporal coherence, it can be termed as a quasi-supercontinuum.
Here, we present an ultrabroadband wavelength-swept source based on a different approach that does not require an additional modulator. For wavelength tuning we employed a microstructured, dispersion-engineered silica fiber, which was pumped by an ultrafast self-modulated laser operating at 1050 nm. The used fiber supported strong Raman self-frequency shift up to 1800 nm and simultaneous generation of a dispersive wave down to 485 nm. By a proper alignment of the pump laser cavity, we could achieve simultaneous mode-locking of the two transverse electromagnetic modes and . Such a regime was investigated previously in Ti:sapphire oscillators and has been termed as total mode-locking, considering concurrent locking of both the longitudinal and transverse modes [24–27]. To the best of our knowledge, this is the first study of total mode-locking in an Yb-doped bulk laser. This mechanism introduced spatial sweeping of the output beam, which consecutively modulated the coupling efficiency to the PCF. Effectively, this induced wavelength sweeping at the PCF output. The frequency of the modulation could be changed between 7 and 21.5 MHz by changing the geometry of the pump laser cavity. By controlling the contribution of the mode, we were able to continuously tune the sweeping bandwidth—eventually covering both the 1300 nm and 1700 nm bioimaging windows. When compared with previously demonstrated solutions, our setup offers not only much broader tunability and higher speed but also reduced complexity and cost, due to the lack of an additional intensity modulator.
Sign up for Photonics Research TOC. Get the latest issue of Photonics Research delivered right to you!Sign up now
2. EXPERIMENTAL SETUP
The experimental setup of the ultrafast wavelength-tunable laser source is presented in Fig. 1(a). The PCF was pumped by a mode-locked oscillator, which delivered nearly transform-limited 85 fs -shaped pulses with an average power up to 290 mW [28]. The home-built laser was pumped by a 1 W single-mode fiber-coupled laser diode and emitted a 14 nm broad spectrum centered at 1049 nm. The autocorrelation trace and the optical spectrum are presented in Fig. 1(b). The repetition frequency was 65 MHz, resulting in a maximum peak power of 46 kW.
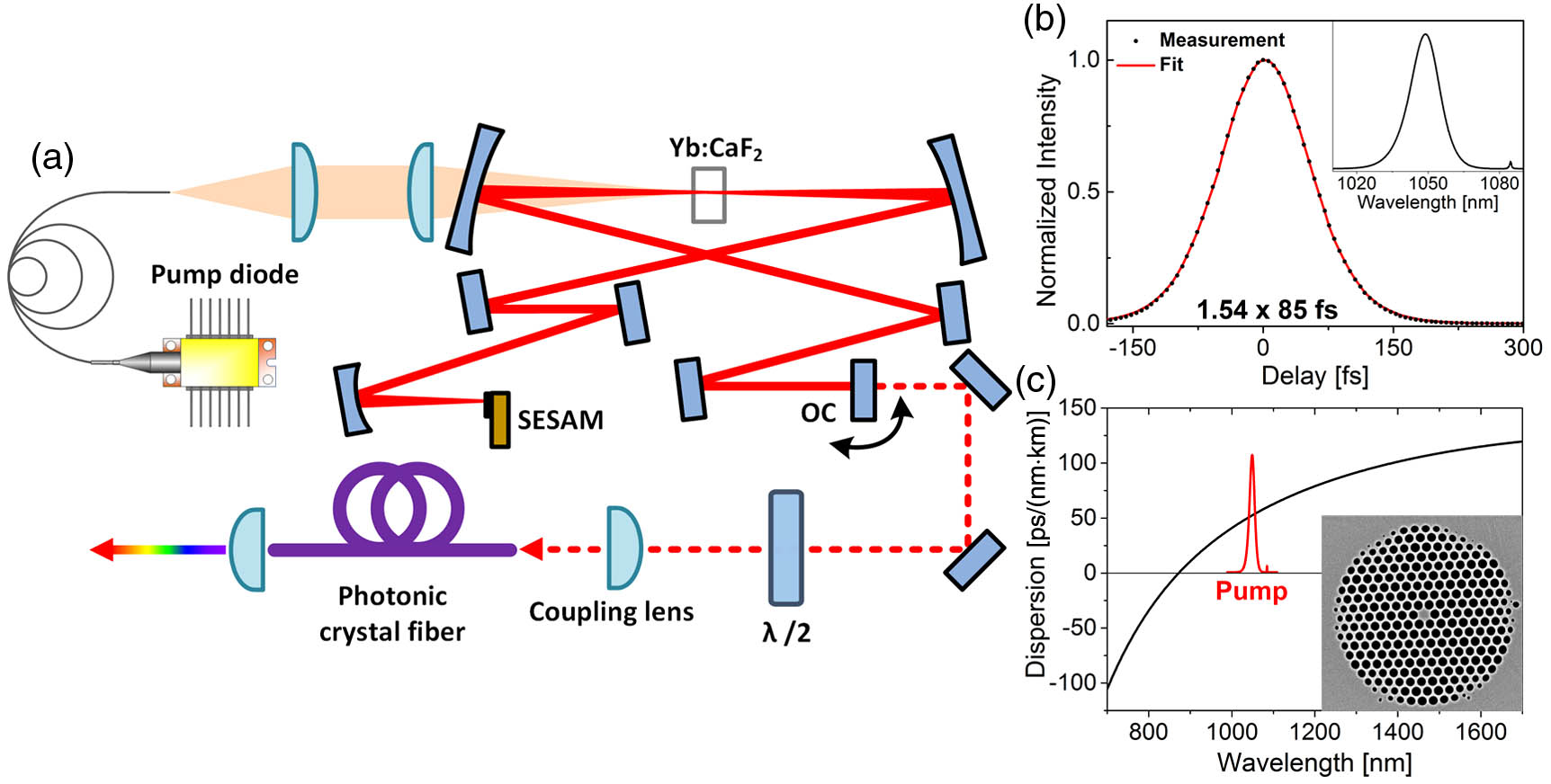
Figure 1.(a) Schematic of the experimental setup: SESAM, semiconductor saturable absorber mirror; OC, output coupler; , half-wave plate. (b) Output characteristics of the mode-locked oscillator: autocorrelation trace with a corresponding fit and (inset) optical spectrum. (c) Dispersion of the PCF with indicated pump spectrum and (inset) scanning electron microscope cross-section image of the PCF.
When correctly aligned, the mode-locked laser emitted a beam with a pure profile. However, we could simultaneously excite a higher mode by slightly tilting the output coupler (OC) end mirror. In such a case, the total electric field is a sum of the fields of the two present TEM modes: Here, is a complex field amplitude associated with a phase-locked superposition of longitudinal modes resulting in a pulsed operation. corresponds to the Hermite–Gaussian function of a mode, which determines the spatial profile of the beam. The contribution of the individual modes is given by a parameter , which is simply a ratio of the mode amplitudes. is a constant phase difference between the two modes. However, the total phase difference varies with time as the frequency of the distinct transverse modes is not the same. The frequency of a longitudinal mode corresponding to a transverse mode is given by , where is the resonator length, and is a parameter associated with the geometry of the cavity and can be derived with ABCD matrix formalism [27]. The time-dependent phase difference between the two fields will introduce a beating resulting in spatial oscillations of the beam profile. This mechanism is illustrated in Fig. 2, which presents the profiles of the and modes as well as the resulting intensity of the total electric field (cross sections with constant ; with arbitrarily chosen ) at and . Figures 2(c) and 2(d) present the same case but with a higher parameter . It is clear that an increase of the mode contribution (i.e., higher ) will result in a stronger modulation (i.e., increased distance between the extreme beam positions). An animation presenting how the beam profile changes with the relative phase difference (with ) is shown in Visualization 1. The sweeping rate is equal to frequency separation between the transverse modes and can be related to the mode-locked repetition frequency . Consequently, it can be controlled by proper changes of the cavity geometry parameters.
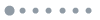
Figure 2.Electric field profiles of the cross sections of and modes (dashed lines) with the resulting normalized intensity of the total electric field (solid lines) at (a), (c) and (b), (d) . The ratio of the mode amplitudes is equal to (a), (b) 0.2 in the upper row and (c), (d) 0.7 in the lower row. For animation, see Visualization 1 ().
The PCF used for spectral shifting had a silica core with a 3.07 μm diameter, which was surrounded by eight rings of air holes. The distance between the holes was equal to 2.47 μm, and the average diameter of the air holes was 1.84 μm. In the experiment, we used a 4 m long piece of the fiber. Figure 1(c) shows the chromatic dispersion curve of the PCF, together with a scanning electron microscope (SEM) image of the end face. The light was coupled to the fiber with an aspheric lens with a focal length of 6.5 mm. Since the PCF is slightly birefringent (due to its elliptical core shape), we employed a half-wave plate to rotate the polarization in order to maximize the frequency shift. The fiber was angle-cleaved (at around 15°) to prevent backreflections, which can disturb stable mode-locked operation of the pump laser.
3. RESULTS
Initially, the pump laser operated in the stable regime with a Gaussian output beam profile. When the light from the oscillator was coupled to the PCF, we could observe a gradual frequency shift of the soliton pulses. For the maximum coupling efficiency of 41%, the central wavelength was shifted up to 1800 nm, and several consecutive higher-order solitons were present. Moreover, the blueshifted part stretched up to 485 nm (with the strongest component at 540 nm) and exhibited a more complicated structure. The entire spectrum emitted from the PCF is shown in Fig. 3(a) together with the beam profile (registered with a scanning slit beam profiler before the coupling lens) and stable pulse train (measured with a fast photodiode after the fiber).
Sign up for Photonics Research TOC. Get the latest issue of Photonics Research delivered right to you!Sign up now
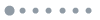
Figure 3.Averaged beam profiles at different regimes with corresponding pulse trains and spectra (in logarithmic scale) registered at the PCF output. The set (a) corresponds to the pump laser operating at a stable, pure Gaussian regime; in (b) we started the modulation by exciting the mode. The modulation effect is more profound in (c), where the contribution of the higher transverse mode is stronger.
Importantly, the achieved coupling efficiency holds only for the pump laser working at the basic transverse mode. If we now consider the movement of the beam off-center of the employed aspheric lens, the coupling efficiency will be reduced. Consequently, spatial sweeping of the beam originating from total mode-locking of the and modes will result in periodic changes of the power coupled to the PCF. Variations of the pump pulse energy propagating along the fiber will be translated to changes in frequency shift of the Raman solitons, i.e., wavelength sweeping.
Consequently, we slightly tilted the end mirror in order to introduce spatial beam sweeping as described in the previous section. Despite the minor change of the beam profile, the modulation of the pulse energy coupled to the PCF is clearly visible in the pulse train [Fig. 3(b)]. In this case, the modulation rate was approximately 6 times lower than the repetition rate of the pump laser and amounted to 11 MHz. This induced wavelength sweeping, which is depicted in the spectrum. It was registered with a standard optical spectrum analyzer, which obviously cannot resolve the pulse-to-pulse spectral deviations and instead measures only a time-averaged spectrum.
We could control the relative amplitudes of the individual transverse modes by changing the tilt of the end mirror. An increase of the tilt led to a growing contribution of the mode, stronger spatial oscillations (see comparison in Fig. 2), and hence to a buildup of the sweeping bandwidth. For example, the longest wavelength component in Fig. 3(b) is centered at 1645 nm and covers 210 nm (between the maxima). However, the bandwidth can be continuously tuned: as we increase the tilt, the distance between the extreme positions of the spatially sweeping beam is also increased, resulting in a larger size of the registered (averaged) beam profile [Fig. 3(c)]. Consequently, the modulation depth is considerably higher, increasing the spectral sweeping range. The components associated with individual Raman solitons overlap, resulting in a quasi-continuous spectrum with a supercontinuum-like shape.
In order to better illustrate the wavelength-sweeping effect, we performed additional measurement with a dispersive Fourier transform (DFT) technique, which enables us to study shot-to-shot spectral dynamics in real time [29]. In the DFT experiment, the output pulses from the PCF were coupled into 1200 m of Corning Vascade S1000 fiber. The temporal trace was observed with a 6 GHz oscilloscope (Agilent DSO90604A Infiniium) coupled with a 12 GHz photodiode (Discovery Semiconductors DSC-50S). Visualization 2 presents one of the measurements: initially the PCF was pumped with a beam, and we could observe three consecutive Raman-shifted solitons. Subsequently, the beam sweeping was started by tilting the mirror. The sweeping magnitude could be easily controlled in a continuous manner by changing the mirror tilt. Finally, the pump laser could be repetitively switched between the stable and modulated regimes.
Once the cavity is aligned for a given modulation depth, the operation and obtained spectral profile are stable. In long-term tests, we have observed the gradual decrease of the pump laser output power (by ), inducing gradual spectral narrowing at the red side of the spectrum. This behavior was observed only for the multi-mode regime, and in the future it can be compensated for by active control of the power of the laser diode pumping the oscillator.
In addition to the modulation depth, its frequency (sweeping rate) can also be controlled. As has been previously demonstrated, the frequency separation between the transverse modes can be changed by modifying the cavity geometry [27]. In Fig. 3, we present the case where it was approximately 6 times smaller than the repetition rate and amounted to 11 MHz. However, by a proper cavity realignment, we were able to tune the modulation frequency. In our case, it was performed by continuous change of the second folding mirror position. Figure 4 depicts the pulse trains measured at the PCF output for modulation frequencies of 7 MHz and 21.5 MHz. Tuning of the sweeping rate introduced minor deviations to the final output spectrum. It is important to note that the modulation frequency does not have to be an integer divisor of the pulse repetition rate. If it is not the case, the pulse train will not be locked to the modulating envelope, resulting in pulse energy deviations between the subsequent sweeping periods. Finally, we were naturally limited by a mode-locked repetition rate of the employed laser source.
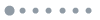
Figure 4.Pulse trains after the PCF at the modulation frequency of (a) 7 MHz and (b) 21.5 MHz.
4. CONCLUSIONS
In summary, we have demonstrated a novel concept for a wavelength-swept source based on total mode-locking of an femtosecond laser. Wavelength tuning was achieved via intensity-dependent SSFS and dispersive wave generation in a highly nonlinear photonic crystal fiber. With a stable pump oscillator, we achieved spectral broadening between 485 and 1800 nm. We could introduce phase-locking of the longitudinal and transverse ( and ) modes simply by tilting the pump laser end mirror. This introduced spatial oscillations of the output beam, which led to modulation of the coupling efficiency to the fiber and effectively induced wavelength sweeping. The sweeping bandwidth can be continuously adjusted by controlling the contribution of the mode (with a mirror tilt). Simultaneously, the sweeping rate could be tuned between 7 and 21.5 MHz by changing the pump laser cavity alignment. Our approach enables us to obtain higher speed and broader tuning range when compared with previously demonstrated solutions. It can operate in both the 1300 nm and 1700 nm bioimaging windows, and it does not require an additional intensity modulator. Finally, this technique can be further developed concerning possible pumping with multimode mode-locked fiber lasers, a field that is currently rapidly developing [30,31].
Acknowledgment
Acknowledgment. M. Kowalczyk acknowledges doctoral fellowships from the National Science Centre (NCN, Poland) and from the Foundation for Polish Science (FNP).
References
[1] P. Herman, B. Maliwal, H. J. Lin, J. Lakowicz. Frequency-domain fluorescence microscopy with the LED as a light source. J. Microsc., 203, 176-181(2001).
[2] M. V. Sarunic, S. Weinberg, J. A. Izatt. Full-field swept-source phase microscopy. Opt. Lett., 31, 1462-1464(2006).
[3] S. R. Chinn, E. A. Swanson, J. G. Fujimoto. Optical coherence tomography using a frequency-tunable optical source. Opt. Lett., 22, 340-342(1997).
[4] M. Wojtkowski. High-speed optical coherence tomography: basics and applications. Appl. Opt., 49, D30-D61(2010).
[5] D. Huang, E. A. Swanson, C. P. Lin, J. S. Schuman, W. G. Stinson, W. Chang, M. R. Hee, T. Flotte, K. Gregory, C. A. Puliafito, J. G. Fujimoto. Optical coherence tomography. Science, 254, 1178-1181(1991).
[6] U. Sharma, E. W. Chang, S. H. Yun. Long-wavelength optical coherence tomography at 1.7 μm for enhanced imaging depth. Opt. Express, 16, 19712-19723(2008).
[7] V. M. Kodach, J. Kalkman, D. J. Faber, T. G. van Leeuwen. Quantitative comparison of the OCT imaging depth at 1300 nm and 1600 nm. Biomed. Opt. Express, 1, 176-185(2010).
[8] S. P. Chong, C. W. Merkle, D. F. Cooke, T. Zhang, H. Radhakrishnan, L. Krubitzer, V. J. Srinivasan. Noninvasive, in vivo imaging of subcortical mouse brain regions with 1.7 μm optical coherence tomography. Opt. Lett., 40, 4911-4914(2015).
[9] C. Chong, T. Suzuki, A. Morosawa, T. Sakai. Spectral narrowing effect by quasi-phase continuous tuning in high-speed wavelength-swept light source. Opt. Express, 16, 21105-21118(2008).
[10] S. Yamashita, M. Asano. Wide and fast wavelength-tunable mode-locked fiber laser based on dispersion tuning. Opt. Express, 14, 9299-9306(2006).
[11] S. Yamashita. Dispersion-tuned swept lasers for optical coherence tomography. IEEE J. Sel. Top. Quantum Electron., 24, 6800109(2018).
[12] R. Huber, M. Wojtkowski, J. G. Fujimoto. Fourier domain mode locking (FDML): a new laser operating regime and applications for optical coherence tomography. Opt. Express, 14, 3225-3237(2006).
[13] F. M. Mitschke, L. F. Mollenauer. Discovery of the soliton self-frequency shift. Opt. Lett., 11, 659-661(1986).
[14] N. Nishizawa, T. Goto. Compact system of wavelength-tunable femtosecond soliton pulse generation using optical fibers. IEEE Photon. Technol. Lett., 11, 325-327(1999).
[15] J. H. Lee, J. Van Howe, C. Xu, X. Liu, S. Member. Soliton self-frequency shift: experimental demonstrations and applications. IEEE J. Sel. Top. Quantum Electron., 14, 713-723(2008).
[16] G. Soboń, T. Martynkien, K. Tarnowski, P. Mergo, J. Sotor. Generation of sub-100 fs pulses tunable from 1700 to 2100 nm from a compact frequency-shifted Er-fiber laser. Photon. Res., 5, 151-155(2017).
[17] G. Soboń, T. Martynkien, D. Tomaszewska, K. Tarnowski, P. Mergo, J. Sotor. All-in-fiber amplification and compression of coherent frequency-shifted solitons tunable in the 1800–2000 nm range. Photon. Res., 6, 368-372(2018).
[18] N. Akhmediev, M. Karlsson. Cherenkov radiation emitted by solitons in optical fibers. Phys. Rev. A, 51, 2602-2607(1995).
[19] G. Chang, L.-J. Chen, F. X. Kärtner. Highly efficient Cherenkov radiation in photonic crystal fibers for broadband visible wavelength generation. Opt. Lett., 35, 2361-2363(2010).
[20] T. Hori, N. Nishizawa, H. Nagai, M. Yoshida, T. Goto. Electronically controlled high-speed wavelength-tunable femtosecond soliton pulse generation using acoustooptic modulator. IEEE Photon. Technol. Lett., 13, 13-15(2001).
[21] M. E. Masip, A. A. Rieznik, P. G. König, D. F. Grosz, A. V. Bragas, O. E. Martinez. Femtosecond soliton source with fast and broad spectral tunability. Opt. Lett., 34, 842-844(2009).
[22] K. Sumimura, T. Ohta, N. Nishizawa. Quasi-super-continuum generation using ultrahigh-speed wavelength-tunable soliton pulses. Opt. Lett., 33, 2892-2894(2008).
[23] K. Sumimura, Y. Genda, T. Ohta, K. Itoh, N. Nishizawa. Quasi-supercontinuum generation using 1.06 μm ultrashort-pulse laser system for ultrahigh-resolution optical-coherence tomography. Opt. Lett., 35, 3631-3633(2010).
[24] D. Auston. Transverse mode locking. IEEE J. Quantum Electron., 4, 420-422(1968).
[25] P. W. Smith. Simultaneous phase‐locking of longitudinal and transverse laser modes. Appl. Phys. Lett., 13, 235-237(1968).
[26] D. Côté, H. M. van Driel. Period doubling of a femtosecond Ti:sapphire laser by total mode locking. Opt. Lett., 23, 715-717(1998).
[27] S. R. Bolton, R. A. Jenks, C. N. Elkinton, G. Sucha. Pulse-resolved measurements of subharmonic oscillations in a Kerr-lens mode-locked Ti:sapphire laser. J. Opt. Soc. Am. B, 16, 339-344(1999).
[28] M. Kowalczyk, A. Major, J. Sotor. High peak power ultrafast Yb:CaF2 oscillator pumped by a single-mode fiber-coupled laser diode. Opt. Express, 25, 26289-26295(2017).
[29] K. Goda, B. Jalali. Dispersive Fourier transformation for fast continuous single-shot measurements. Nat. Photonics, 7, 102-112(2013).
[30] L. G. Wright, D. N. Christodoulides, F. W. Wise. Spatiotemporal mode-locking in multimode fiber lasers. Science, 358, 94-97(2017).
[31] W. Fu, L. G. Wright, P. Sidorenko, S. Backus, F. W. Wise. Several new directions for ultrafast fiber lasers [Invited]. Opt. Express, 26, 9432-9463(2018).