
- Photonics Research
- Vol. 10, Issue 9, 2229 (2022)
Abstract
1. INTRODUCTION
With the exploration and development of wide bandgap semiconductors, solid-state solar-blind ultraviolet (SBUV) photodetectors have attracted more and more research attention [1–3]. The SBUV detection, however, sets out unique requirements for the “5S” key parameters (i.e., high sensitivity, high spectral selectivity, high signal-to-noise ratio, high speed, and high stability) of usual high-performance photodetectors. Since the SBUV signal (200–280 nm) is easily attenuated during propagation and is usually very weak, high sensitivity is particularly important for the photodetectors. Sensitivity of
Generally, high internal gain is a prerequisite to achieve high sensitivity of photodetectors. The main types of high-gain photodetectors are avalanche photodiodes (APDs), unipolar field-effect phototransistors (FEPTs), and bipolar heterojunction phototransistors (HPTs). Despite the multiplication gain of more than
The spontaneous polarization effect is an intrinsic property of a number of compound semiconductors, originating from the inversion asymmetry of the crystal structure [21]. In wurtzite AlGaN, in addition to spontaneous polarization, piezoelectric polarization due to the presence of biaxial stress also contributes significantly to macroscopic polarization [22]. The strong polarization effect induces a large electric field in heteroepitaxial nitrides, which not only has a significant impact on the device characteristics [23,24], but also opens up a way for the development of new structures for optoelectronic devices.
Sign up for Photonics Research TOC. Get the latest issue of Photonics Research delivered right to you!Sign up now
In this work, an
2. EXPERIMENTS
The
After the epitaxial growth, interdigital electrodes with Ti/Al/Ni/Au (15/80/20/60 nm) metal stacks were deposited by electron-beam evaporation and thermally annealed in pure
Figure 1.Schematic diagram of
The structural properties of the epitaxial samples were characterized by high-resolution X-ray diffraction (HR-XRD) measurements (Brucker D8 Discovery). The transmittance spectrum in the wavelength range of 200–800 nm was measured using a UV-visible spectrophotometer (Shimadzu UV2550). A Kelvin probe force microscope (KPFM) (Brucker Dimension Edge) was used to determine the surface potential of
3. RESULTS AND DISCUSSION
First, the structural and optical properties of the epitaxial structure with a 70-nm-thick
Figure 2.(a) (0002) plane and (
Second, to compare the depletion effect of the polarization electric field on the electrons in n-type
Figures 3(a) and 3(b) show the I–V characteristics of the photodetectors under dark and irradiation conditions. The dark current (
Figure 3.Dark and illuminated
As a key parameter for high-sensitivity photodetectors, optical gain (
Next, the photoresponse characteristics of the FCSD phototransistor were further investigated. Figure 4(a) shows the spectral responses of the detector at different bias voltages. A sharp cutoff can be found at the wavelength of
Figure 4.Photoresponse characteristics of the FCSD phototransistor. (a) Spectral responses at different bias voltages. (b) Bias dependence of responsivity at 240 and 280 nm. (c) Time and irradiation intensity dependence of the response current under 5-V bias, in which the irradiation uses periodic DUV illumination with periodic on/off times of 10/20 s. (d) Transient responses to the 213-nm pulse signal with an optical power density of
Figure 4(c) gives the time and irradiation intensity dependence of the response current under 5 V bias. One can see that the detector clearly recognizes 260-nm periodic irradiation of different intensities with excellent stability and reproducibility. A steep rise and fall of the response current can be observed with the on and off of DUV illumination. Furthermore, the transient response of the device was measured. The load resistor used in the measurement is 50 Ω. Figure 4(d) shows the impulse responses at 20-V bias. The rise time from 10% to 90% of the response peak is 537.5 ps, while the decay time from 90% to 10% is 3.1 μs. The FWHM of a single impulse response is 130 ns, which is one of the highest response speeds of various SBUV detectors reported so far. Our calculations show that the rise time is approximate to the electron transit time (
The specific detectivity (
Furthermore, the dependence of the response current on the incident optical power density was investigated. Figure 5(a) shows the I–V curves at DUV irradiation intensity ranging from 0 to
Figure 5.(a)
According to the power law, the incident optical power dependence can be expressed as
In stage II, a linear fitting to the power dependence yields
To clarify the mechanism behind the high-performance detection, we analyzed the photoresponse process of the detector. A schematic diagram of the transport processes of photogenerated carriers in the
Figure 6.(a) Schematic diagram of the neutral conductive path formed in the n-AlGaN channel of FCSD phototransistor under DUV irradiation due to the action of virtual photogate (separation of photogenerated electrons and holes). (b) Schematic diagram of energy band under dark and on illumination conditions.
Generally, the photoresponse of the FCSD field-effect phototransistor due to the photovoltage
The
On the other hand, the
Accordingly, the theoretical
The above photoresponse mechanism shows that the
A comparative chart is presented in Table 1, which summarizes the key characteristic parameters of SBUV photodetectors in this work and in previous reports based on different materials [37–47]. Compared with the previously reported detectors, our FCSD phototransistor exhibits the best comprehensive performance, including extremely low
Summary of Key Characteristic Parameters of Solar-Blind UV Photodetectors Based on Different Materials in This Work and Previous Literatures
Material | Detector Type | Detectivity (Jones) | PDCR | Reference | ||
---|---|---|---|---|---|---|
MSM | – | [ | ||||
MSM | 8.9 | – | [ | |||
APD | [ | |||||
PEDOT: | p-n heterojunction | 2.6 | [ | |||
MSM | 230 | [ | ||||
FE | [ | |||||
FE | [ | |||||
Microflake FE | [ | |||||
p-i-n | 0.211 | – | [ | |||
Schottky | 0.033 | – | – | [ | ||
HFEPT | 4.4/591 | [ | ||||
MSM | – | [ | ||||
HPT | 360 | – | 0.97/12.6 | [ | ||
APD | 202.8 | – | [ | |||
FCSD-phototransistor | This work |
MSM, metal semiconductor metal; FE, field effect; HFEPT, heterojunction field-effect phototransistor.
Johnson noise limited
Shot noise limited
Johnson and shot noise limited
4. CONCLUSION
In summary, two-terminal FCSD AlGaN/AlN solar-blind phototransistors with superior performance and a simple epitaxial structure have been fabricated. The full-channel self-depletion of the n-type conductive
References
[1] C. Xie, X. T. Lu, X. W. Tong, Z. X. Zhang, F. X. Liang, L. Liang, L. B. Luo, Y. C. Wu. Recent progress in solar-blind deep-ultraviolet photodetectors based on inorganic ultrawide bandgap semiconductors. Adv. Funct. Mater., 29, 1806006(2019).
[2] Q. Cai, H. You, H. Guo, J. Wang, B. Liu, Z. Xie, D. Chen, H. Lu, Y. Zheng, R. Zhang. Progress on AlGaN-based solar-blind ultraviolet photodetectors and focal plane arrays. Light Sci. Appl., 10, 94(2021).
[3] U. Varshney, N. Aggarwal, G. Gupta. Current advances in solar-blind photodetection technology: using Ga2O3 and AlGaN. J. Mater. Chem. C, 10, 1573-1593(2022).
[4] A. Hirano, C. Pernot, M. Iwaya, T. Detchprohm, H. Amano, I. Akasaki. Demonstration of flame detection in room light background by solar-blind AlGaN PIN photodiode. Phys. Status Solidi A, 188, 293-296(2001).
[5] C. Coetzer, S. Groenewald, W. Leuschner. An analysis of the method for determining the lowest sensitivity of solar-blind ultraviolet corona cameras. International SAUPEC/RobMech/PRASA Conference, 1-6(2020).
[6] Z. Xu, B. M. Sadler. Ultraviolet communications: potential and state-of-the-art. IEEE Commun. Mag., 46, 67-73(2008).
[7] H. Wu, W. Wu, H. Zhang, Y. Chen, Z. Wu, G. Wang, H. Jiang. All AlGaN epitaxial structure solar-blind avalanche photodiodes with high efficiency and high gain. Appl. Phys. Express, 9, 052103(2016).
[8] B. Liu, D. Chen, H. Lu, T. Tao, Z. Zhuang, Z. Shao, W. Xu, H. Ge, T. Zhi, F. Ren, J. Ye, Z. Xie, R. Zhang. Hybrid light emitters and UV solar-blind avalanche photodiodes based on III-nitride semiconductors. Adv. Mater., 32, 1904354(2020).
[9] A. Yoshikawa, Y. Yamamoto, T. Murase, M. Iwaya, T. Takeuchi, S. Kamiyama, I. Akasaki. High-photosensitivity AlGaN-based UV heterostructure-field-effect-transistor-type photosensors. Jpn. J. Appl. Phys., 55, 05FJ04(2016).
[10] K. Wang, X. Qiu, Z. Lv, Z. Song, H. Jiang. Ultrahigh detectivity, high-speed and low-dark current AlGaN solar-blind heterojunction field-effect phototransistors realized using dual-float-photogating effect. Photon. Res., 10, 111-119(2021).
[11] Y. Liu, L. Du, G. Liang, W. Mu, Z. Jia, M. Xu, Q. Xin, X. Tao, A. Song. Ga2O3 field-effect-transistor-based solar-blind photodetector with fast response and high photo-to-dark current ratio. IEEE Electron Device Lett., 39, 1696-1699(2018).
[12] C. Chen, X. Zhao, X. Hou, S. Yu, R. Chen, X. Zhou, P. Tan, Q. Liu, W. Mu, Z. Jia, G. Xu, X. Tao, S. Long. High-performance β-Ga2O3 solar-blind photodetector with extremely low working voltage. IEEE Electron Device Lett., 42, 1492-1495(2021).
[13] M. I. Pintor-Monroy, M. G. Reyes-Banda, C. Avila-Avendano, M. A. Quevedo-Lopez. Tuning electrical properties of amorphous Ga2O3 thin films for deep UV phototransistors. IEEE Sens. J., 21, 14807-14814(2021).
[14] X. Sun, Z. Wang, H. Gong, X. Chen, Y. Zhang, Z. Wang, X. Yu, F. Ren, H. Lu, S. Gu, Y. Zheng, R. Zhang, J. Ye. M-plane α-Ga2O3 solar-blind detector with record-high responsivity-bandwidth product and high-temperature operation capability. IEEE Electron Device Lett., 43, 541-544(2022).
[15] P. Reddy, M. Hayden Breckenridge, Q. Guo, A. Klump, D. Khachariya, S. Pavlidis, W. Mecouch, S. Mita, B. Moody, J. Tweedie, R. Kirste, E. Kohn, R. Collazo, Z. Sitar. High gain, large area, and solar blind avalanche photodiodes based on Al-rich AlGaN grown on AlN substrates. Appl. Phys. Lett., 116, 081101(2020).
[16] Z. Shao, H. Yu, Y.-S. Liu, X. Yang, D. Chen, B. Liu, H. Lu, R. Zhang, Y. Zheng. Different I-V behaviors and leakage current mechanisms in AlGaN solar-blind ultraviolet avalanche photodiodes. ACS Appl. Electron. Mater., 2, 2716-2720(2020).
[17] L. Zhang, S. Tang, C. Liu, B. Li, H. Wu, H. Wang, Z. Wu, H. Jiang. Demonstration of solar-blind Al
[18] D. Chen, D. Li, G. Zeng, F. C. Hu, Y. C. Li, Y. C. Chen, X. X. Li, J. Tang, C. Shen, N. Chi, D. W. Zhang, H. L. Lu. GaN-based micro-light-emitting diode driven by a monolithic integrated ultraviolet phototransistor. IEEE Electron Device Lett., 43, 80-83(2022).
[19] A. M. Armstrong, B. Klein, A. A. Allerman, E. A. Douglas, A. G. Baca, M. H. Crawford, G. W. Pickrell, C. A. Sanchez. Visible-blind and solar-blind detection induced by defects in AlGaN high electron mobility transistors. J. Appl. Phys., 123, 114502(2018).
[20] J. Z. Li, J. Y. Lin, H. X. Jiang, M. Asif Khan, Q. Chen. Persistent photoconductivity in a two-dimensional electron gas system formed by an AlGaN/GaN heterostructure. J. Appl. Phys., 82, 1227-1230(1997).
[21] C. Wood, D. Jena. Polarization Effects in Semiconductors: From Ab Initio Theory to Device Applications(2007).
[22] F. Bernardini, V. Fiorentini, D. Vanderbilt. Spontaneous polarization and piezoelectric constants of III-V nitrides. Phys. Rev. B, 56, R10024(1997).
[23] O. Ambacher, R. Dimitrov, M. Stutzmann, B. E. Foutz, M. J. Murphy, J. A. Smart, J. R. Shealy, N. G. Weimann, K. Chu, M. Chumbes, B. Green, A. J. Sierakowski, W. J. Schaff, L. F. Eastman. Role of spontaneous and piezoelectric polarization induced effects in group-III nitride based heterostructures and devices. Phys. Status Solidi B, 216, 381-389(1999).
[24] C. X. Ren. Polarisation fields in III-nitrides: effects and control. Mater. Sci. Technol., 32, 418-433(2016).
[25] Z. Li, P. Shao, Y. Wu, G. Shi, T. Tao, Z. Xie, P. Chen, Y. Zhou, X. Xiu, D. Chen, B. Liu, K. Wang, Y. Zheng, R. Zhang, T. Lin, L. Wang, H. Hirayama. Plasma assisted molecular beam epitaxy growth mechanism of AlGaN epilayers and strain relaxation on AlN templates. Jpn. J. Appl. Phys., 60, 075504(2021).
[26] J. D. Wei, S. F. Li, A. Atamuratov, H. H. Wehmann, A. Waag. Photoassisted Kelvin probe force microscopy at GaN surfaces: the role of polarity. Appl. Phys. Lett., 97, 172111(2010).
[27] S. M. Sze, K. K. Ng. Physics of Semiconductor Devices(2007).
[28] V. S. N. Chava, B. G. Barker, A. Balachandran, A. Khan, G. Simin, A. B. Greytak, M. V. S. Chandrashekhar. High detectivity visible-blind SiF4 grown epitaxial graphene/SiC Schottky contact bipolar phototransistor. Appl. Phys. Lett., 111, 243504(2017).
[29] J. He, H. Liu, C. Huang, Y. Jia, K. Li, A. Mesli, R. Yang, Y. He, Y. Dan. Analytical transient responses and gain-bandwidth products of low-dimensional high-gain photodetectors. ACS Nano, 15, 20242-20252(2021).
[30] B. Chen, W. Y. Jiang, J. Yuan, A. L. Holmes, B. M. Onat. Demonstration of a room-temperature InP-based photodetector operating beyond 3 μm. IEEE Photon. Technol. Lett., 23, 218-220(2011).
[31] Z. Lv, Y. Guo, S. Zhang, Q. Wen, H. Jiang. Polarization engineered InGaN/GaN visible-light photodiodes featuring high responsivity, bandpass response, and high speed. J. Mater. Chem. C, 9, 12273-12280(2021).
[32] S. Rathkanthiwar, A. Kalra, S. V. Solanke, N. Mohta, R. Muralidharan, S. Raghavan, D. N. Nath. Gain mechanism and carrier transport in high responsivity AlGaN-based solar blind metal semiconductor metal photodetectors. J. Appl. Phys., 121, 164502(2017).
[33] D. A. Neamen. Semiconductor Physics and Devices: Basic Principles(2003).
[34] Y. Taniyasu, M. Kasu. Polarization property of deep-ultraviolet light emission from C-plane AlN/GaN short-period superlattices. Appl. Phys. Lett., 99, 251112(2011).
[35] X. Chen, Y. Xu, D. Zhou, S. Yang, F.-F. Ren, H. Lu, K. Tang, S. Gu, R. Zhang, Y. Zheng, J. Ye. Solar-Blind photodetector with high avalanche gains and bias-tunable detecting functionality based on metastable phase α-Ga2O3/ZnO isotype heterostructures. ACS Appl. Mater. Interfaces, 9, 36997-37005(2017).
[36] Z. Z. Bandić, P. M. Bridger, E. C. Piquette, T. C. McGill. Minority carrier diffusion length and lifetime in GaN. Appl. Phys. Lett., 72, 3166-3168(1998).
[37] W. E, M. Li, D. Meng, Y. Cheng, W. Fu, P. Ye, Y. He. High-performance amorphous BeZnO-alloy-based solar-blind ultraviolet photodetectors on rigid and flexible substrates. J. Alloys Compd., 831, 154819(2020).
[38] M. M. Fan, K. W. Liu, Z. Z. Zhang, B. H. Li, X. Chen, D. X. Zhao, C. X. Shan, D. Z. Shen. High-performance solar-blind ultraviolet photodetector based on mixed-phase ZnMgO thin film. Appl. Phys. Lett., 105, 011117(2014).
[39] H. Wang, H. Chen, L. Li, Y. Wang, L. Su, W. Bian, B. Li, X. Fang. High responsivity and high rejection ratio of self-powered solar-blind ultraviolet photodetector based on PEDOT:PSS/β-Ga2O3 organic/inorganic p-n junction. J. Phys. Chem. Lett., 10, 6850-6856(2019).
[40] Y. Qin, L. Li, X. Zhao, G. S. Tompa, H. Dong, G. Jian, Q. He, P. Tan, X. Hou, Z. Zhang, S. Yu, H. Sun, G. Xu, X. Miao, K. Xue, S. Long, M. Liu. Metal-semiconductor-metal ε-Ga2O3 solar-blind photodetectors with a record-high responsivity rejection ratio and their gain mechanism. ACS Photon., 7, 812-820(2020).
[41] Y. Qin, H. Dong, S. Long, Q. He, G. Jian, Y. Zhang, X. Zhou, Y. Yu, X. Hou, P. Tan, Z. Zhang, Q. Liu, H. Lv, M. Liu. Enhancement-mode β-Ga2O3 metal-oxide-semiconductor field-effect solar-blind phototransistor with ultrahigh detectivity and photo-to-dark current ratio. IEEE Electron Device Lett., 40, 742-745(2019).
[42] S. Yu, X. Zhao, M. Ding, P. Tan, X. Hou, Z. Zhang, W. Mu, Z. Jia, X. Tao, G. Xu, S. Long. High-detectivity β-Ga2O3 microflake solar-blind phototransistor for weak light detection. IEEE Electron Device Lett., 42, 383-386(2021).
[43] A. Kalra, S. Rathkanthiwar, R. Muralidharan, S. Raghavan, D. N. Nath. Polarization-graded AlGaN solar-blind p-i-n detector with 92% zero-bias external quantum efficiency. IEEE Photon. Technol. Lett., 31, 1237-1240(2019).
[44] V. Adivarahan, G. Simin, G. Tamulaitis, R. Srinivasan, J. Yang, M. A. Khan, M. S. Shur, R. Gaska. Indium-silicon co-doping of high-aluminum-content AlGaN for solar blind photodetectors. Appl. Phys. Lett., 79, 1903-1905(2001).
[45] A. Yoshikawa, S. Ushida, M. Iwaya, T. Takeuchi, S. Kamiyama, I. Akasaki. Influence of trap level on an Al0.6Ga0.4N/Al0.5Ga0.5N metal-semiconductor-metal UV photodetector. Jpn. J. Appl. Phys., 58, SCCC26(2019).
[46] Q. Wen, C. Wang, X. Qiu, Z. Lv, H. Jiang. Significant performance improvement of AlGaN solar-blind heterojunction phototransistors by using Na2S solution based surface treatment. Appl. Surf. Sci., 591, 153144(2022).
[47] T. Tut, M. Gokkavas, A. Inal, E. Ozbay. Al
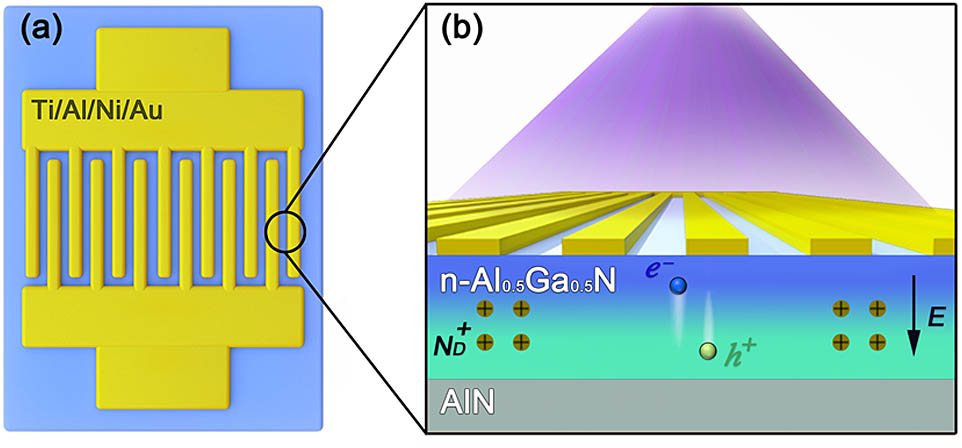
Set citation alerts for the article
Please enter your email address