Author Affiliations
1Key Laboratory for Micro/Nano Optoelectronic Devices of Ministry of Education & Hunan Provincial Key Laboratory of Low-Dimensional Structural Physics and Devices, School of Physics and Electronics, Hunan University, Changsha 410082, China2State Key Laboratory on Integrated Optoelectronics, College of Electronic Science & Engineering, Jilin University, Changchun 130012, China3Changchun Observatory, National Astronomical Observatories, Chinese Academy of Sciences, Changchun 130117, China4Hunan Key Laboratory for Micro-Nano Energy Materials and Devices, Xiangtan University, Xiangtan 411105, China5e-mail: qings@jlu.edu.cn6Institute for Lasers, Photonics, Biophotonics, University at Buffalo, State University of New York, Buffalo, New York 14260, USAshow less
Abstract
Mid-infrared pulsed lasers operating around the 3?μm wavelength regime are important for a wide range of applications including sensing, spectroscopy, imaging, etc. Despite the recent advances in technology, the lack of a nonlinear optical modulator operating in the mid-infrared regime remains a significant challenge. Here, we report the third-order nonlinear optical response of gold nanorods (GNRs) ranging from 800?nm to the mid-infrared regime (2810?nm) enabled by their size and overlapping behavior-dependent longitudinal surface plasmon resonance. In addition, we demonstrate a wavelength-tunable -doped fluoride fiber laser modulated by GNRs, which can deliver pulsed laser output, with the pulse duration down to 533?ns, tunable wavelength ranging from 2760.2 to 2810.0?nm, and spectral 3?dB bandwidth of about 1?nm. The experimental results not only validate the GNRs’ robust mid-infrared nonlinear optical response, but also manifest their application potential in high-performance broadband optoelectronic devices.1. INTRODUCTION
Nonlinear optical material plays an important role for optical communication, optical sensing, biophotonics, etc. While various nonlinear optical materials have been developed, the lack of nonlinear optical materials with broadband nonlinear response, especially extending to the mid-infrared spectral range, remains a significant challenge, since they are highly required for some important and unique applications in laser surgery, laser radar, gas sensing, etc. [1]. When it comes to the mid-infrared regime, nonlinear optical materials will play a very important role to manipulate the light intracavity or extracavity. If it was introduced into the laser cavity, the nonlinear optical materials can act as a nonlinear pulse modulator, i.e., saturable absorber (SA), which can transit between different absorption states within a fast time scale, to modulate the laser system to deliver the pulsed laser. In addition, the laser can deliver flexible tunable output with a deliberate laser design, which will be very important for the specific applications of mid-infrared light. Inspired by the requirements to fabricate a cost-effective SA with a broadband, ultrafast, and tunable optical response, substantial progress has been made by using semiconductor saturable absorber mirrors (SESAMs), , and two-dimensional (2D) materials, such as graphene, , black phosphorus, , as the nonlinear modulator to modulate the mid-infrared pulse laser in the past few years [2–17]. However, the SESAMs are expensive and exhibit limited operation bandwidth. For 2D materials, the defect-prone exfoliation and transfer processes inevitably lead to poor repeatability and reliability, and it has been challenging to tune the intrinsic nonlinear optical response in these material systems. Therefore, improving the laser performance of current mid-infrared pulsed lasers critically depends on the availability of a capable optical modulator, preferably with a broadband nonlinear response and high modulation depth [1–3].
Gold nanorods (GNRs), an outstanding plasmonic metal nanomaterial, have sparked growing interest for their applications in biomedicine and optics motivated by their biocompatibility, easy fabrication, and peculiar optical properties [18–23]. Surface plasmon plays an important role for the excellent physical properties of GNRs, which can lead to many charming optical phenomena by interacting with the incident electromagnetic field. GNRs have two surface plasmon resonance (SPR) bands: the transverse SPR (TSPR) band and the longitudinal SPR (LSPR) band. The TSPR band is always situated in the visible region, whereas the LSPR band could be tuned flexibly by varying the aspect ratio of the GNRs [24,25]. Moreover, the nonlinear optical response of GNRs, especially the property of saturable absorption around the LSPR band with the Z-scan technique, has been investigated recently [26]. At the same time, the large third-order nonlinear response and ultrafast recovery time on a picosecond time scale have been reported as well [27–29]. The most intriguing aspect is that the LSPR effect can significantly enhance the optical nonlinearity without sacrificing the ultrafast response [20]. Based on their excellent nonlinear properties, GNRs as SAs have been utilized in passively -switched/mode-locked fiber lasers and solid-state lasers [29–36]. However, the above results mainly focused on the visible or near-infrared (NIR) wavelength. As mentioned above, the LSPR band of GNRs can be tuned by varying the aspect ratio of the GNRs. Meanwhile, the GNRs also have a large third-order nonlinear response and ultrafast recovery time on a picosecond time scale. Hence, the interests for GNRs as the SA in mid-infrared pulse lasers have been paralleled by the development of novel nonlinear optical materials at visible and NIR wavelengths.
Here, we investigated the third-order nonlinear optical response of the GNRs in the mid-infrared wavelength regime enabled by the LSPR of GNRs. Moreover, we realized a pulsed fiber laser modulated by GNRs. A stable -switched pulse laser with a 50 nm tuning range from 2760.2 to 2810.0 nm and a spectral 3 dB bandwidth of about 1 nm has been obtained. When the laser operates at 2786 nm, the shortest pulse width of 533 ns and maximum pulse energy of 3.3 μJ can be delivered.
Sign up for Photonics Research TOC. Get the latest issue of Photonics Research delivered right to you!Sign up now
2. PREPARATION AND CHARACTERIZATION OF GOLD NANORODS
GNRs in this experiment were synthesized by the seed-mediated growth method [37,38]. The seed solution for GNRs was prepared as reported previously [27]. 5 mL of 0.034 mol/L aqueous solution was mixed with 20 mL of 2 mol/L CTAB solution in a beaker. The mixture solution was stirred at 60°C for about 15 min. Then 1 mL of 0.0005 mol/L fresh solution was injected into the above seed solution. The color of the solution changed from yellow to dark brown. The seed solution was kept for 1 h at 60°C and then used for the synthesis of GNRs. The growth solution was prepared by adding 0.03 mol CTAB and 0.0007 mol ortho-hydroxybenzoic acid into 31.25 mL deionized water in a beaker, and the solution was vigorously stirred at 60°C. Then 1 mL of 0.004 mol/L AgNO3 solution, 0.156 mL of 0.00065 mol/L ascorbic acid aqueous solution, and 31.25 mL of 0.03375 mol/L HAuCl4 aqueous solution were added into the above-mentioned solution. The color of the solution turned to orange at once and then became colorless. 0.226 mL of 12 mol/L HCl was used to reduce the pH value below 7.0. Finally, 0.2 mL of the seed solution was injected into the growth solution to initiate the growth of the GNRs. The final solution was kept at room temperature for 8 h, and the GNR solution was obtained. Figure 1(a) shows the transmission electron microscopy (TEM) image of prepared GNRs. Here, a large quantity of rod-shaped nanoparticles was obtained, and only a small amount of the spherical nanoparticles exists in the GNR sample. The diameters of these GNRs were about 25 nm, and the aspect ratios were in the range of 4.5 to 8, as shown in Fig. 1(b). Most of the GNRs () have aspect ratios of 6–7. The inset of Fig. 1(a) shows the photograph of the aqueous solution of GNRs. Then the GNR film was formed by casting the GNRs aqueous solution onto a flat substrate, followed by a slow drying procedure at room temperature. The absorption spectrum of GNRs (the absorption of substrate has been deducted) was characterized with an ultraviolet (UV)-visible NIR spectrophotometer (UV-3600 Shimadzu), as shown in Fig. 2(a). The TSPR absorption peak was recorded at 546 nm, while the LSPR peak emerged at 1050 nm with the LSPR band extended to 3200 nm. In general, the limitation of the LSPR absorption peak at a long wavelength is about 1.2 μm for the single GNR, which was synthesized by using seed-mediated processes [39]. Recently, both the experimental and theoretical results have proved that the LSPR absorption peak of GNRs can be tuned from 1 to 3 μm by using directed end-to-end assembly approach, and the absorption peak of assembled GNRs was also linearly related to the templet chain length [38–45]. Thus, the SPR band of GNRs that extends over 3000 nm may be caused by overlapping of GNRs with different aspect ratios or the self-assembly of GNRs. We have also modeled the LSPR characteristics of concatenated GNRs numerically by the finite-difference time-domain (FDTD) method. Figure 2(b) shows the LSPR absorption characteristics of one GNR, two GNRs, three GNRs, and four GNRs concatenated. We could see that the LSPR absorption peaks redshift with the increase in the number of GNRs concatenated. Moreover, the absorption cross section of the LSPR was gradually increased.
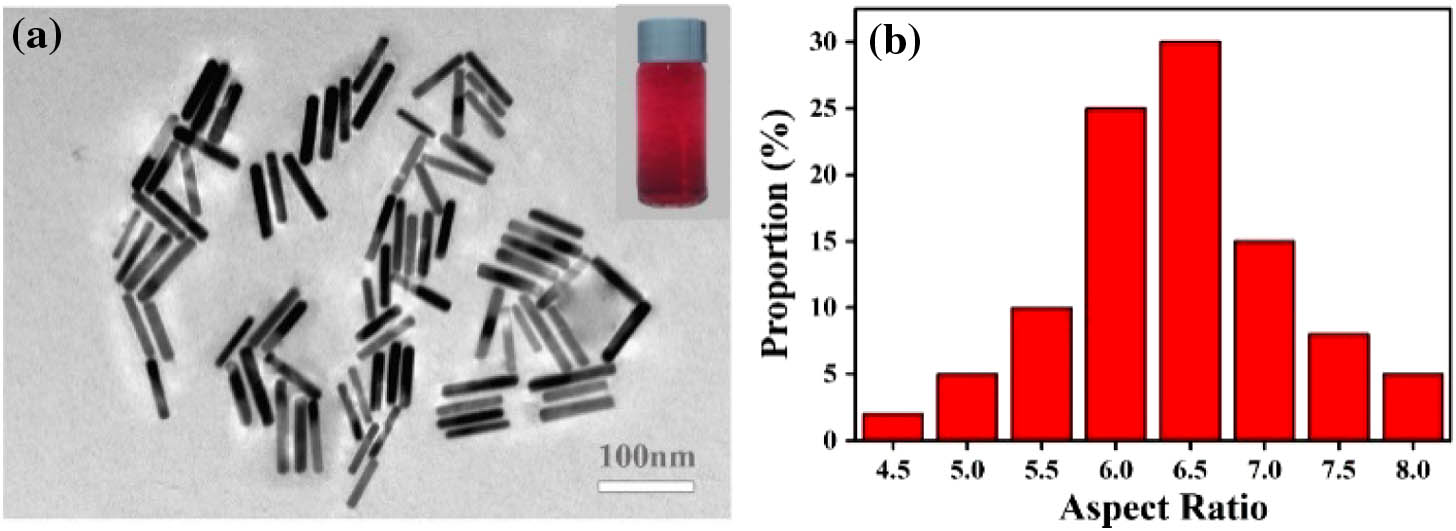
Figure 1.(a) TEM image and (b) aspect ratio distribution of GNRs. The inset of (a) shows the photograph of the GNR solution.
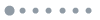
Figure 2.(a) Absorption spectrum of GNRs from 400 to 3200 nm. (b) The FDTD simulation results of the absorption cross section of one, two, three, and four GNRs concatenated.
Although the linear absorption band of GNRs near 3 μm is lower than that around absorption peaks at 546 and 1050 nm, the nonlinear optical response of GNRs near 3 μm cannot be ignored. The broadband nonlinear absorption characteristics of the GNRs were verified by using ultrafast laser sources with different wavelengths (780 nm ultrafast lasers with repetition rate 80 MHz, pulse width 100 fs; 1560 nm ultrafast lasers with repetition rate 80 MHz, pulse width 100 fs; 1930 nm ultrafast lasers with repetition rate 32.3 MHz, pulse width 2.8 ps) based on the open-aperture Z-scan technique. Figures 3(a)–3(c) have shown the nonlinear measurement results of GNRs. The experiment results were fitted by using the nonlinear absorption model [46,47]. The modulation depth of the SA of GNRs can be obtained by fitting the experiment data with Eq. (1). The fitted modulation depths are 13.1%, 12.9%, and 6.2% for 780 nm, 1560 nm, and 1930 nm, respectively.To investigate the nonlinear absorption of the prepared GNRs’ SA in mid-infrared regime, the nonlinear absorption of GNRs was investigated by the power-dependent transmittance measurement system. The pulsed laser source is an in-house nanosecond pulse mid-infrared optical parametric oscillator (OPO) system emitting at [48]. In order to avoid the strong absorption of the quartz substrate at the mid-infrared wavelength, the aqueous solution of the GNRs was drop-cast onto a flat substrate. In addition, we have cast the aqueous solution three times after the GNRs aqueous solution dried at room temperature to increase sample thickness and hence the absorbance. By fitting the experimental results, we obtained a modulation depth of 25.1%, as can be seen in Fig. 3(d). These results suggest that the fabricated GNRs’ SA has an ultrabroadband nonlinear optical absorption property, which can be used to modulate the broadband laser from the NIR to mid-infrared wavelength range.
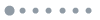
Figure 3.Nonlinear saturable absorption measurements of GNRs at 780 nm, 1560 nm, 1930 nm, and .
3. RESULTS AND DISCUSSION
The experiment setup of the wavelength-tunable passively -switched fiber laser with GNRs as the SA is shown in Fig. 4. A 976 nm fiber-coupled laser diode with a core diameter of 105 μm and NA of 0.22 was the pump source. The pump beam was collimated by (Thorlabs, LA1951-B) and focused by (Thorlabs, LA5763) on the end of a 3.9 m double-clad -doped ZBLAN fiber (FiberLabs, Japan). is a 45° dichroic mirror with high reflectivity at the lasing wavelength and high transmission at 976 nm. The dopant concentration of -doped ZBLAN fiber is 6 mol. %, and the core diameter is 18 μm with an NA of 0.12. The fiber inner cladding is octagonal with a diameter of 330 μm across the circular cross section. The front face of fiber was perpendicularly cleaved to improve by 4% Fresnel reflection, and the rear face was cleaved at an angle of 8°. In order to eliminate the influence of heat, both ends of the fiber were mounted in a heat sink with a V-groove. The laser beam output from the rear face was collimated by an antireflection coated objective lens (Thorlabs, LA5315). The GNRs were located in the confocal arrangement (, Thorlabs, LA5315; , Thorlabs, LA5817) with an optimized position. The wavelength-tunable system is composed of a plane mirror (with reflectivity of 99% for the lasing wavelength) and volume Bragg grating (VBG). The central wavelength of the VBG (Optigrate Corp.) is 2950 nm. The pulse trains were measured by an infrared HgCdTe detector and a 4 GHz digital oscilloscope. An optical spectrum analyzer (Princeton Instruments, Acton SP2300) with a scanning resolution of 0.1 nm was used to measure the output wavelength. In order to obtain maximum output power, the operating wavelength of the fiber laser was tuned to 2786 nm, which is approximately the wavelength of free oscillation.
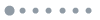
Figure 4.Experiment schematic of a tunable passively -switched fiber laser using gold nanorods as the saturable absorber.
The passively -switched pulse could be observed when the incident pump power of the laser diode increased to 2.76 W. The output pulse laser characteristics of the passively -switched fiber laser are shown in Fig. 5. The output spectrum of the -switched fiber laser operating at 2786 nm has been shown in Fig. 5(a). We can see that the center wavelength of the -switched laser is located at 2786 nm. Figure 5(b) shows that the output power and pulse energy increase linearly with an increase of the pump power. A maximum output power of 172 mW and maximum pulse energy of 3.3 μJ can be obtained at a pump power of 5.6 W. Figure 5(c) depicts that the pulse full width at half-maximum (FWHM) was gradually decreased from 3.7 μs to 533 ns, and the pulse repetition rate was increased from 27.8 to 52.1 kHz as a function of incident pump power, which presents a typical feature of passively -switched lasers. Figure 5(d) shows the experimental result of the radio frequency at the maximum incident pump power, which shows a signal-to-noise ratio of 38 dB. The -switched pulse trains were recorded at different incident powers, and a typical single pulse profile is shown in Fig. 6. We can see that the pulse repetition rate gradually increased, and at the same time, the pulse width gradually decreased as the tunable potential of the fiber laser, which is highly desired for some applications, such as laser scalpel and noninvasive medical diagnosis. Due to the thermal accumulation of the GNRs, the -switched pulse train will become unstable and noisier at higher repetition rates. By maintaining the incident pump power of 5.6 W, the tunable wavelength output can be realized with the VBGs, which is an ideal candidate for wavelength selection and spectral linewidth narrowing [48]. As depicted in Fig. 7(a), the wavelength can be tuned from 2760.2 nm to 2810.0 nm, and the spectral 3 dB bandwidth can be narrowed to be about 1 nm. Figure 7(b) has shown the relationship of output power and wavelength. We can see that the maximum output power is around 170 mW with the wavelength located at 2786.0 nm. Remarkably, the passive -switching could be always observed during the wavelength-tunable process. The pulsed fiber laser has been realized based on different nonlinear optical modulators. To elucidate the merits of GNRs, we have summarized the nonlinear optical parameters and the output characteristics of the passively -switched fiber lasers using different SAs, as shown in Table 1. From the table, it can be seen that the GNRs can exhibit high modulation depth, and the fiber laser modulated by GNRs can deliver comparable pulse duration as the fiber laser with graphene and [2–5]. One can believe that the shorter pulse width with GNRs can be anticipated by optimizing the doping concentration or the fiber length of fiber. Compared to the crystal, GNRs have some peculiar advantages, such as low cost and easy fabrication. On the other hand, the pulse energy and pulse peak power of the fiber laser with GNRs are approximately comparable to those from other reported results [6–8]. The comparison strongly indicates that GNRs can act as a high-performance SA.
Q-switcher | Nonlinear Parameters | Laser Parameters | Refs. |
λ (μm) | Δα (%) | Δτ (ns) | E (μJ) | Pp(W) |
Fe2+:ZnSe | 2.8 | / | 370 | 2 | 5.3 | [6] |
Graphene | 2.8 | / | 400 | 6.4 | 16.1 | [4] |
Black phosphorus | 2.78 | 15 | 1180 | 7.7 | 6.5 | [5] |
Cu2−xS nanocrystal | 2.77 | / | 750 | 2.36 | 3.1 | [3] |
Bi2Te3 | 2.8 | 40.6 | 1300 | 9.3 | 7.1 | [6] |
Gold nanorods | 2.78 | 21.3 | 533 | 3.3 | 6.2 | This work |
Table 1. Comparison of Output Characteristics of Passively -switched Fiber Lasers Using Various Saturable Absorbers
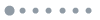
Figure 5.(a) Output spectrum of the -switched fiber laser operating at 2786 nm. (b) Output power and pulse energy as a function of incident pump power. (c) Repetition rate and pulse width as a function of incident pump power. (d) Radio-frequency spectrum.
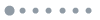
Figure 6.-switched pulse train at different output powers and the typical single pulse profile from the fiber laser.
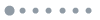
Figure 7.Output characteristics of the tunable passively -switched fiber laser: (a) output spectrum of tunable passively -switched fiber laser; (b) output power as a function of wavelength with an incident pump power of 5.6 W.
4. CONCLUSION
In summary, we demonstrated the ultrabroadband nonlinear optical absorption properties of GNRs from 800 nm to 2810 nm using Z-scan and power-dependent transmittance measurement systems. Owing to the overlapping of GNRs with different aspect ratios and the self-assembly of GNRs, a robust and high-performance tunable mid-infrared passively -switched fiber laser has been demonstrated based on the tunable LSPR band and broadband nonlinear response of GNRs. A stable pulse train with a 50 nm tuning range from 2760.2 to 2810.0 nm and a spectral 3 dB bandwidth of 1 nm were obtained. The tunable LSPR band and nonlinear response of the GNRs will deepen the understanding of LSPR behavior and provide new opportunities for the high-performance mid-infrared nonlinear optoelectronic devices.
References
[1] S. D. Jackson. Towards high-power mid-infrared emission from a fibre laser. Nat. Photonics, 6, 423-431(2012).
[2] C. H. Zhu, F. Q. Wang, Y. F. Meng, X. F. Yuan, F. X. Xiu, H. Y. Luo, Y. Z. Wang, J. F. Li, X. J. Lv, L. Liang, Y. B. Xu, J. F. Liu, C. Zhang, Y. Shi, R. Zhang, S. N. Zhu. A robust and tuneable mid-infrared optical switch enabled by bulk Dirac fermions. Nat. Commun., 8, 14111(2017).
[3] Q. B. Guo, Y. H. Yao, Z. C. Luo, Z. P. Qin, G. Q. Xie, M. Liu, J. Kang, S. A. Zhang, G. Bi, X. F. Liu, J. R. Qiu. Universal near-infrared and mid-infrared optical modulation for ultrafast pulse generation enabled by colloidal plasmonic semiconductor nanocrystals. ACS Nano, 10, 9463-9469(2016).
[4] S. Tokita, M. Murakami, S. Shimizu, M. Hashida, S. Sakabe. Graphene Q-switching of a 3 μm Er: ZBLAN fiber laser. Advanced Solid-State Lasers Congress, AF2A.9(2013).
[5] Z. P. Qin, G. Q. Xie, H. Zhang, C. J. Zhao, P. Yuan, S. C. Wen, L. J. Qian. Black phosphorus as saturable absorber for the Q-switched Er3+: ZBLAN fiber laser at 2.8 μm. Opt. Express, 23, 24713-24718(2015).
[6] C. Wei, X. S. Zhu, R. A. Norwood, N. Peyghambarian. Passively Q-switched 2.8 μm nanosecond fiber laser. IEEE Photon. Technol. Lett., 24, 1741-1744(2012).
[7] P. H. Tang, M. Wu, Q. K. Wang, L. L. Miao, B. Huang, J. Liu, C. J. Zhao, S. C. Wen. 2.8-μm pulsed Er3+: ZBLAN fiber laser modulated by topological insulator. IEEE Photon. Technol. Lett., 28, 1573-1576(2016).
[8] M. Q. Fan, T. Li, S. Z. Zhao, G. Q. Li, H. Y. Ma, X. H. Gao, C. Kränkel, G. Huber. Watt-level passively Q-switched Er: Lu2 O3 laser at 2.84 μm using MoS2. Opt. Lett., 41, 540-543(2016).
[9] P. H. Tang, Z. P. Qin, J. Liu, C. J. Zhao, G. Q. Xie, S. C. Wen, L. J. Qian. Watt-level passively mode-locked Er3+-doped ZBLAN fiber laser at 2.8 μm. Opt. Lett., 40, 4855-4858(2015).
[10] J. F. Li, H. Y. Luo, Y. L. He, Y. Liu, L. Zhang, K. M. Zhou, A. G. Rozhin, S. K. Turistyn. Semiconductor saturable absorber mirror passively Q-switched 2.97 μm fluoride fiber laser. Laser Phys. Lett., 11, 065102(2014).
[11] Z. K. Liu, H. R. Mu, S. Xiao, R. B. Wang, Z. T. Wang, W. W. Wang, Y. J. Wang, X. X. Zhu, K. Y. Lu, H. Zhang, S. T. Lee, Q. L. Bao, W. L. Ma. Pulsed lasers employing solution‐processed plasmonic Cu3–xP colloidal nanocrystals. Adv. Mater., 28, 3535-3542(2016).
[12] K. Yin, B. Zhang, L. Li, T. Jiang, X. F. Zhou. Soliton mode-locked fiber laser based on topological insulator Bi2Te3 nanosheets at 2 μm. Photon. Res., 3, 72-76(2015).
[13] W. Q. Yang, J. Hou, B. Zhang, R. Song, Z. J. Hou. Semiconductor saturable absorber mirror passively Q-switched fiber laser near 2 μm. Appl. Opt., 51, 5664-5667(2012).
[14] Y. H. Lin, S. H. Lin, Y. C. Chi, C. L. Wu, C. H. Cheng, W. H. Tseng, J. H. He, C. I. Wu, C. K. Lee, G. R. Lin. Using n- and p-type Bi2Te3 topological insulator nanoparticles to enable controlled femtosecond mode-locking of fiber lasers. ACS Photon., 2, 481-490(2015).
[15] Y. R. Wang, P. Lee, B. T. Zhang, Y. H. Sang, J. L. He, H. Liu, C. K. Lee. Optical nonlinearity engineering of a bismuth telluride saturable absorber and application of a pulsed solid state laser therein. Nanoscale, 9, 19100-19107(2018).
[16] D. Li, H. Xue, Y. D. Wang, S. Aksimsek, N. Chekurov, W. Kim, C. F. Li, J. Riikonen, F. W. Ye, Q. Dai, Z. Y. Ren, J. T. Bai, T. Hasan, H. Lipsanen, Z. P. Sun. Active synchronization and modulation of fiber lasers with a graphene electro-optic modulator. Opt. Lett., 43, 3497-3500(2018).
[17] X. T. Kong, B. Bai, Q. Dai. Graphene plasmon propagation on corrugated silicon substrates. Opt. Lett., 40, 1-4(2015).
[18] A. Kinkhabwala, Z. Yu, S. Fan, Y. Avlasevich, K. Müllen, W. E. Moerner. Large single-molecule fluorescence enhancements produced by a bowtie nanoantenna. Nat. Photonics, 3, 654-657(2009).
[19] G. A. Wurtz, R. Pollard, W. Hendren, G. P. Wiederrechat, D. J. Gosztola, V. A. Podolskiy, A. V. Zayats. Nanostructured materials for photon detection. Nat. Nanotechnol., 6, 107-111(2010).
[20] M. Kauranen, A. V. Zayats. Nonlinear plasmonics. Nat. Photonics, 6, 737-748(2012).
[21] H. Y. Wu, H. C. Chu, T. J. Kuo, C. L. Kuo, M. H. Huang. Seed-mediated synthesis of high aspect ratio gold nanorods with nitric acid. Chem. Mater., 17, 6447-6451(2005).
[22] L. Vigderman, B. P. Khanal, E. R. Zubarev. Functional gold nanorods: synthesis, self‐assembly, and sensing applications. Adv. Mater., 24, 4811-4841(2012).
[23] P. Anger, P. Bharadwaj, L. Novotny. Enhancement and quenching of single-molecule fluorescence. Phys. Rev. Lett., 96, 113002(2006).
[24] K. Maximova, A. Aristov, M. Sentis, A. V. Kabashin. Size-controllable synthesis of bare gold nanoparticles by femtosecond laser fragmentation in water. Nanotechnology, 26, 065601(2015).
[25] H. I. Elim, J. Yang, J. Y. Lee, J. Mi, W. Ji. Observation of saturable and reverse-saturable absorption at longitudinal surface plasmon resonance in gold nanorods. Appl. Phys. Lett., 88, 083107(2006).
[26] J. O. Banska, M. Gordel, R. Kolkowski, K. Matczyszyn, M. Samoc. Third-order nonlinear optical properties of colloidal gold nanorods. J. Phys. Chem. C, 116, 13731-13737(2012).
[27] S. Link, C. Burda, M. B. Mohamed, B. Nikoobakht, M. A. El-Sayed. Femtosecond transient-absorption dynamics of colloidal gold nanorods: shape independence of the electron-phonon relaxation time. Phys. Rev. B, 61, 6086-6090(2006).
[28] Z. Kang, Y. Xu, L. Zhang, Z. X. Jia, L. Liu, D. Zhao, Y. Feng, G. S. Qin, W. P. Qin. Passively mode-locking induced by gold nanorods in erbium-doped fiber lasers. Appl. Phys. Lett., 103, 041105(2013).
[29] T. Jiang, Y. Xu, Q. Tian, L. Liu, Z. Kang, R. Y. Yang, G. S. Qin, W. P. Qin. Passively Q-switching induced by gold nanocrystals. Appl. Phys. Lett., 101, 151122(2012).
[30] H. T. Huang, M. Li, L. Wang, X. Liu, D. Y. Shen, D. Y. Tang. Gold nanorods as single and combined saturable absorbers for a high-energy Q-switched Nd:YAG solid-state laser. IEEE Photon. J., 7, 14501210(2015).
[31] H. Zhang, J. Liu. Gold nanobipyramids as saturable absorbers for passively Q-switched laser generation in the 1.1 μm region. Opt. Lett., 41, 1150-1152(2016).
[32] S. X. Wang, Y. X. Zhang, J. Xin, X. F. Liu, H. H. Yu, A. D. Lieto, M. Tonelli, T. C. Sum, H. J. Zhang, Q. H. Xiong. Nonlinear optical response of Au nanorods for broadband pulse modulation in bulk visible lasers. Appl. Phys. Lett., 107, 161103(2015).
[33] D. D. Wu, J. Ping, Z. P. Cai, J. Weng, Z. Q. Luo, N. Chen, H. Y. Xu. Gold nanoparticles as a saturable absorber for visible 635 nm Q-switched pulse generation. Opt. Express, 23, 24071-24076(2015).
[34] X. D. Wang, Z. C. Luo, M. Liu, R. Tang, A. P. Luo, W. C. Xu. Wavelength-switchable femtosecond pulse fiber laser mode-locked by silica-encased gold nanorods. Laser Phys. Lett., 13, 045101(2016).
[35] Z. Kang, X. Y. Guo, Z. X. Jia, Y. Xu, L. Liu, D. Zhao, G. S. Qin, W. P. Qin. Gold nanorods as saturable absorbers for all-fiber passively Q-switched erbium-doped fiber laser. Opt. Mater. Express, 3, 1986-1991(2013).
[36] B. Nikoobakht, M. A. El-Sayed. Preparation and growth mechanism of gold nanorods (NRs) using seed-mediated growth method. Chem. Mater., 15, 1957-1962(2003).
[37] X. Ye, L. Jin, H. Caglayan, J. Chen, G. Xing, C. Zheng, V. Doan-Nguyen, Y. Kang, N. Engheta, C. R. Kagan, C. B. Murray. Improved size-tunable synthesis of monodisperse gold nanorods through the use of aromatic additives. ACS Nano, 6, 2804-2817(2012).
[38] K. Park, S. Biswas, S. Kanel, D. Nepal, R. A. Vaia. Engineering the optical properties of gold nanorods: independent tuning of surface plasmon energy, extinction coefficient, and scattering cross section. J. Phys. Chem. C, 118, 5918-5926(2014).
[39] J. Fontana, R. Nita, N. Charipar, J. Naciri, K. Park, A. Dunkelberger, J. Owrustsky, A. Qique, R. Vaia, B. Ratna. Widely tunable infrared plasmonic nanoantennas using directed assembly. Adv. Opt. Mater., 5, 1700335(2017).
[40] J. Fontana, N. Charipar, S. R. Flom, J. Naciri, A. Piqué, B. R. Ratna. Rise of the charge transfer plasmon: programmable concatenation of conductively linked gold nanorod dimers. ACS Photon., 3, 904-911(2016).
[41] G. González-Rubio, J. González-Izquierdo, L. Bares, G. Tardajos, A. Rivera, T. Altantzis, S. Bals, O. Pena-Rodríguez, A. Guerrero-Martínez, L. M. Liz-Marzán. Femtosecond laser-controlled tip-to-tip assembly and welding of gold nanorods. Nano Lett., 15, 8282-8288(2015).
[42] L. O. Herrmann, V. K. Valev, C. Tserkezis, J. S. Barnard, S. Kasera, O. A. Scherman, J. Aizpurua, J. J. Baumberg. Threading plasmonic nanoparticle strings with light. Nat. Commun., 5, 4568(2014).
[43] K. Liu, A. Ahmed, S. Chung, K. Sugikawa, G. Wu, Z. Nie, R. Gordon, E. Kumacheva. In situ plasmonic counter for polymerization of chains of gold nanorods in solution. ACS Nano, 7, 5901-5910(2013).
[44] P. Pramod, K. G. Thomas. Plasmon coupling in dimers of Au nanorods. Adv. Mater., 20, 4300-4305(2008).
[45] M. Maldonado, H. T. M. C. M. Baltar, A. S. L. Gomes, R. Vaia, K. Park, J. Che, M. Hsiao, C. B. de Araújo, A. Baev, P. N. Prasad. Coupled-plasmon induced optical nonlinearities in anisotropic arrays of gold nanorod clusters supported in a polymeric film. J. Appl. Phys., 121, 143103(2017).
[46] M. Sheik-Bahae, A. A. Said, T. H. Wei, D. J. Hagan, E. W. Van Stryland. Sensitive measurement of optical nonlinearities using a single beam. IEEE J. Quantum Electron., 26, 760-769(1990).
[47] J. Liu, P. Tang, Y. Chen, C. Zhao, D. Shen, S. Wen, D. Fan. Highly efficient tunable mid-infrared optical parametric oscillator pumped by a wavelength locked, Q-switched Er: YAG laser. Opt. Express, 23, 20812-20819(2015).
[48] P. H. Tang, J. Liu, B. Huang, C. W. Xu, C. J. Zhao, S. C. Wen. Stable and wavelength-locked Q-switched narrow-linewidth Er: YAG laser at 1645 nm. Opt. Express, 23, 11037-11042(2015).