
- Photonics Research
- Vol. 10, Issue 1, 183 (2022)
Abstract
1. INTRODUCTION
The linear Doppler effect describes a phenomenon that electromagnetic waves or sound waves reflected from a moving object will cause a frequency shift, which has been extensively utilized to measure the linear velocity of an object [1]. Analogously, when light is reflected or emitted from a rotating object, it will undergo a frequency shift, which is the rotational version of Doppler effect [2]. The rotational Doppler effect, associated with photon spin angular momentum, was originally observed and analyzed by Garetz
It is worth noting that infrared remote sensing may be more advantageous in certain applications, such as infrared monitoring in the military realm [18], non-destructive and reagent-free biological detection [19–21], remote sensing of urban climate [22], and ocean remote sensing [23,24]. However, suffering from exorbitant prices, relatively low quantum efficiency, severe dark current, and requiring additional cryogenic cooling, the development of infrared detection is seriously affected [25]. Moreover, it is difficult to achieve simple and efficient image detection in the mid-infrared regime with existing detector technologies [26,27]. To circumvent this constraint, ultrasensitive infrared detection can be expected with nonlinear frequency conversion from the infrared light field to the visible spectral region by leveraging the high sensitivity of visible single-photon counting modules [25,28–32]. This strategy has been demonstrated to build all-optical upconversion imaging systems for few-photon-level full two-dimensional infrared imaging [33]. Here, via second-harmonic generation (SHG), we extend this scheme to measure the rotational Doppler shifts from the infrared detected light to visible with a high-efficiency single-photon detector. Actually, a clever scheme has been devised by Li and coworkers to report the rotational Doppler effect in nonlinear optics, in which the frequency shifts were imparted by the rotating nonlinear crystal itself [34,35]. Instead, we rotate one fundamental wave (FW) by illuminating it onto a spinning object while preparing the other FW to carry the desired OAM superpositions and thus enabling the detection of frequency shifts and angular velocity of rotating objects from SHG light.
2. METHOD
The Laguerre–Gaussian (LG) modes, as one kind of typical OAM eigenmode, are conceived as a set of orthogonal and complete basis vectors in high-dimensional Hilbert space. In the cylindrical coordinate
Sign up for Photonics Research TOC. Get the latest issue of Photonics Research delivered right to you!Sign up now
Figure 1.OAM mode spectra
The mathematical picture of eigenmode decomposition offers us a more intuitive understanding on the physical origin of rotational Doppler effect. Assume that the rotating object has a constant angular velocity
It can be seen clearly from Eq. (3) that, as a result of rotation, an additional time-dependent and OAM-related phase variation of
In order to extract the rotational Doppler signals of the infrared light
3. EXPERIMENTS
In our experimental implementation, as shown in Fig. 2, the horizontally polarized light beam derived from a near-infrared 1064 nm laser (Cobolt, Rumba) is expanded and collimated by a telescope, and then it is directed to illuminate a computer-controlled spatial light modulator (SLM, Hamamatsu, X10468-7). The SLM is divided into two regions, each of which has a pixel array of
Figure 2.Experimental setup for upconversion detection of rotational Doppler effect. Insets are the typical holograms displayed in Regions I and II of SLM, respectively.
Subsequently, we use another
In our first set of experiments, we simulate a rotating object of three-leaf clover by using a computer-generation hologram in SLM. For the rotating clover, we set
Figure 3.Experimental results for a rotating clover. (a) and (b) OAM-dependent photon counts (
Without loss of generality, we further consider other objects of more complex structures, such as a snowflake and a cartoon pattern. In our experiment, both patterns are set to rotate at the same constant angular velocity of 4 deg/s. For the snowflake of Figs. 4(a) and 4(c), we can see that the dominant Doppler signals of SHG light appear at the measured OAM superpositions of
Figure 4.Experimental results for a rotating snowflake and a cartoon pattern, respectively. (a) and (b) OAM-dependent photon counts (
4. CONCLUSION
In conclusion, based on frequency upconversion, we have developed a new scheme for detecting the angular velocities of rotating objects via SHG. We have used one infrared FW to probe a rotating object and amplified the tiny angular velocity by the OAM numbers of the other FW as a result of transferring the rotational frequency shifts from the infrared to the visible region at low photon-count rates. Besides, the measured power spectra of Doppler signals also reflect the geometric symmetry of the rotating objects. Also, the periodically poled crystals, e.g., periodically-poled potassium titanyl phosphate (PPKTP), can be a better candidate to enable strong photon–photon interaction and to realize high-efficiency frequency conversion [39]. Thus, infrared monitoring and remote sensing even at the few-photon level will become more feasible in the future studies.
Acknowledgment
Acknowledgment. The authors thank Dr. Tong Liu from Space Engineering University for helpful technical discussions.
References
[1] C. Doppler. Über das farbige Licht der Doppelsterne und einigeranderer Gestirne des Himmels(1842).
[2] M. Padgett. Electromagnetism: like a speeding watch. Nature, 443, 924-925(2006).
[3] B. A. Garetz, S. Arnold. Variable frequency shifting of circularly polarized laser radiation via a rotating half-wave retardation plate. Opt. Commun., 31, 1-3(1979).
[4] J. Courtial, K. Dholakia, D. A. Robertson, L. Allen, M. J. Padgett. Measurement of the rotational frequency shift imparted to a rotating light beam possessing orbital angular momentum. Phys. Rev. Lett., 80, 3217-3219(1998).
[5] J. Courtial, D. A. Robertson, K. Dholakia, L. Allen, M. J. Padgett. Rotational frequency shift of a light beam. Phys. Rev. Lett., 81, 4828-4830(1998).
[6] M. P. Lavery, F. C. Speirits, S. M. Barnett, M. J. Padgett. Detection of a spinning object using light’s orbital angular momentum. Science, 341, 537-540(2013).
[7] L. Marrucci. Spinning the Doppler effect. Science, 341, 464-465(2013).
[8] S. Barreiro, J. W. R. Tabosa, H. Failache, A. Lezama. Spectroscopic observation of the rotational Doppler effect. Phys. Rev. Lett., 97, 113601(2006).
[9] H. Luo, S. Wen, W. Shu, Z. Tang, Y. Zou, D. Fan. Rotational Doppler effect in left-handed materials. Phys. Rev. A, 78, 033805(2008).
[10] O. Korech, U. Steinitz, R. J. Gordon, I. S. Averbukh, Y. Prior. Observing molecular spinning via the rotational Doppler effect. Nat. Photonics, 7, 711-714(2013).
[11] H. Zhou, D. Fu, J. Dong, P. Zhang, X. Zhang. Theoretical analysis and experimental verification on optical rotational Doppler effect. Opt. Express, 24, 10050-10056(2016).
[12] H. L. Zhou, D. Z. Fu, J. J. Dong, P. Zhang, D. X. Chen, X. L. Cai, F. L. Li, X. L. Zhang. Orbital angular momentum complex spectrum analyzer for vortex light based on the rotational Doppler effect. Light Sci. Appl., 6, e16251(2017).
[13] W. Zhang, J. Gao, D. Zhang, Y. He, T. Xu, R. Fickler, L. Chen. Free-space remote sensing of rotation at the photon-counting level. Phys. Rev. Appl., 10, 044014(2018).
[14] Y. Zhai, S. Fu, C. Yin, H. Zhou, C. Gao. Detection of angular acceleration based on optical rotational Doppler effect. Opt. Express, 27, 15518-15527(2019).
[15] S. Qiu, T. Liu, Z. Li, C. Wang, Y. Ren, Q. Shao, C. Xing. Influence of lateral misalignment on the optical rotational Doppler effect. Appl. Opt., 58, 2650-2655(2019).
[16] S. Qiu, T. Liu, Y. Ren, Z. Li, C. Wang, Q. Shao. Detection of spinning objects at oblique light incidence using the optical rotational Doppler effect. Opt. Express, 27, 24781-24792(2019).
[17] W. Zhang, D. Zhang, X. Qiu, L. Chen. Quantum remote sensing of the angular rotation of structured objects. Phys. Rev. A, 100, 043832(2019).
[18] R. D. Hudson, J. W. Hudson. The military applications of remote sensing by infrared. Proc. IEEE, 63, 104-128(1975).
[19] A. Barth. Infrared spectroscopy of proteins. BBA-Bioenergetics, 1767, 1073-1101(2007).
[20] S. Türker-Kaya, C. W. Huck. A review of mid-infrared and near-infrared imaging: principles, concepts and applications in plant tissue analysis. Molecules, 22, 168(2017).
[21] J. Shi, T. T. Wong, Y. He, L. Li, R. Zhang, C. S. Yung, J. Hwang, K. Maslov, L. V. Wang. High-resolution, high-contrast mid-infrared imaging of fresh biological samples with ultraviolet-localized photoacoustic microscopy. Nat. Photonics, 13, 609-615(2019).
[22] Q. Weng. Thermal infrared remote sensing for urban climate and environmental studies: methods, applications, and trends. ISPRS J. Photogramm., 64, 335-344(2009).
[23] M. Wang. Remote sensing of the ocean contributions from ultraviolet to near-infrared using the shortwave infrared bands: simulations. Appl. Opt., 46, 1535-1547(2007).
[24] M. Wang, W. Shi. Estimation of ocean contribution at the MODIS near-infrared wavelengths along the east coast of the US: two case studies. Geophys. Res. Lett., 32, L17708(2005).
[25] M. Mrejen, Y. Erlich, A. Levanon, H. Suchowski. Multicolor time-resolved upconversion imaging by adiabatic sum frequency conversion. Laser Photon. Rev., 14, 2000040(2020).
[26] K. J. Kubarych, M. Joffre, A. Moore, N. Belabas, D. M. Jonas. Mid-infrared electric field characterization using a visible charge-coupled-device-based spectrometer. Opt. Lett., 30, 1228-1230(2005).
[27] M. Vollmer, K.-P. Möllmann. Infrared Thermal Imaging: Fundamentals, Research and Applications(2010).
[28] B. Klein, E. Plis, M. N. Kutty, N. Gautam, A. Albrecht, S. Myers, S. Krishna. Varshni parameters for InAs/GaSb strained layer superlattice infrared photodetectors. J. Phys. D, 44, 075102(2011).
[29] R. H. Hadfield. Single-photon detectors for optical quantum information applications. Nat. Photonics, 3, 696-705(2009).
[30] H. Dong, H. Pan, Y. Li, E. Wu, H. Zeng. Efficient single-photon frequency upconversion at 1.06 μm with ultralow background counts. Appl. Phys. Lett., 93, 071101(2008).
[31] R. T. Thew, H. Zbinden, N. Gisin. Tunable upconversion photon detector. Appl. Phys. Lett., 93, 071104(2008).
[32] C. Pedersen, E. Karamehmedović, J. S. Dam, P. Tidemand-Lichtenberg. Enhanced 2D-image upconversion using solid-state lasers. Opt. Express, 17, 20885-20890(2009).
[33] K. Huang, X. Gu, H. Pan, E. Wu, H. Zeng. Few-photon-level two-dimensional infrared imaging by coincidence frequency upconversion. Appl. Phys. Lett., 100, 151102(2012).
[34] K. F. Li, J. H. Deng, X. Liu, G. Li. Observation of rotational Doppler effect in second harmonic generation in reflection mode. Laser Photon. Rev., 12, 1700204(2018).
[35] G. Li, T. Zentgraf, S. Zhang. Rotational Doppler effect in nonlinear optics. Nat. Phys., 12, 736-740(2016).
[36] L. Torner, J. P. Torres, S. Carrasco. Digital spiral imaging. Opt. Express, 13, 873-881(2005).
[37] R. W. Boyd, B. R. Masters. Nonlinear Optics(2008).
[38] F. Bouchard, N. H. Valencia, F. Brandt, R. Fickler, M. Huber, M. Malik. Measuring azimuthal and radial modes of photons. Opt. Express, 26, 31925-31941(2018).
[39] R. Tang, X. Li, W. Wu, H. Pan, H. Zeng, E. Wu. High efficiency frequency upconversion of photons carrying orbital angular momentum for a quantum information interface. Opt. Express, 23, 9796-9802(2015).
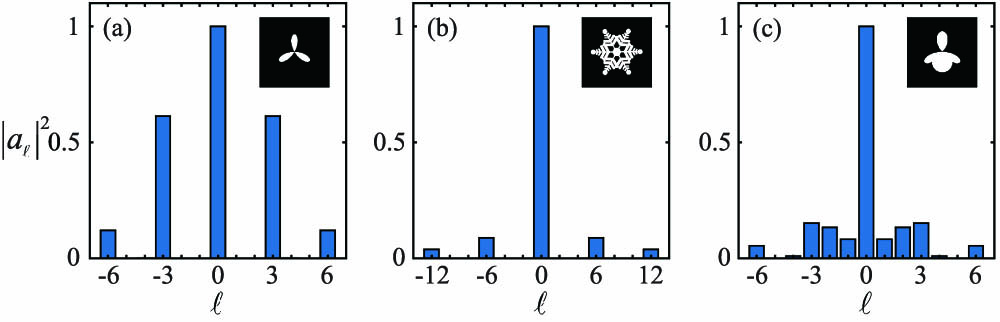
Set citation alerts for the article
Please enter your email address