
- Photonics Research
- Vol. 10, Issue 7, 1594 (2022)
Abstract
1. INTRODUCTION
On-chip integrated multicolor microlasers have been intensively studied owing to their practical applications ranging from miniaturized coherent light source, optical sensing, to visible light communication [1–7]. Compared with the combination of several microlasers, the white-light microlaser from a single nanomaterial is more attractive for on-chip integration [8–16]. In 2015, Fan
Benefiting from their intrinsic features of plentiful energy levels and long decay lifetimes, lanthanide (
2. RESULTS AND DISCUSSION
The controlled growth of
Sign up for Photonics Research TOC. Get the latest issue of Photonics Research delivered right to you!Sign up now
Figure 1.Characterization of
The chemical composition of the glass film can be verified according to the energy-dispersive X-ray spectroscopy (EDS), UV-vis, and photoluminescence (PL) results. Only Si, O, Ca, and Yb elements can be detected from the EDS measurement [Fig. 2(a)]; however, there are characteristic emitting peaks associated with
Figure 2.Structural characterization of
To understand the crystallization of the glass matrix, we carried out thermogravimetry/differential thermal analysis (TG/DTA) and XRD measurements. The amorphous-to-crystalline phase transition was also consistently recorded in TEM results (Fig. 3). Together with the exothermic/endothermic peaks in the DT profile, the TG curve of xerogel in Fig. 2(d) shows four stages of weight loss in air, which can be ascribed to the evaporation of water and organic solvent between 25°C and 110°C, the burnout of excess acetic acid and ethanol between 110°C and 260°C, the complete combustion of polyester via the hydrolysis reaction of tetraethyl orthosilicate (TEOS) and acetates between 260°C and 400°C, and the crystallization of the cubic
Figure 3.Crystallization characteristics. TEM images of the films annealed at (a) room temperature, (b) 400°C, (c) 800°C, and (d) 1000°C, respectively. Size distribution of the observed NCs by counting more than 100 particles is also indicated in (c) and (d). The scale bars are 20, 20, 20, and 50 nm, respectively.
Accordingly, we have explored the dependence of surface quality on the heating rates during the multistep calcination. On the basis of the TG/DTA results, a group of
Figure 4.Evolution of surface roughness. Photographs of
An extensive optical characterization was performed to validate white-light emission behavior of
Figure 5.Optical characterization of
As predicted, the decreasing concentration of
Figure 6.White and full-color tunable photoluminescence. Emission spectra from glass films containing (a)
Then, the possibilities to support white-light emission from the luminescent films were studied from a series of
To confirm this assumption, the
Figure 7.Fabrication of chip-scale
Figure 8.Pure
The microcavities were first characterized in the 1550 nm band with a tapered fiber waveguide, as shown in the left inset of Fig. 9(a) [44,49]. A concise survey on the dependence of the
The lasing characterization of the as-prepared microtoroid [Figs. 9(b)–9(e)] was performed by the free-space excitation. Generally, a pulsed 980 nm laser (6 ns, 10 Hz) from a 355 nm frequency-tripled
The spectral evolution of RGB emissions with the increase of pumping power is depicted in Figs. 9(c)–9(e). Only broadband emission peaks are observed at low power density. Then, periodic peaks maxima at 653, 545, and 475 nm sequentially occur and quickly dominate the emission spectra as the pumping power increases. Owing to the occurrence of stimulated emission, the FWHMs of these peaks collapse to less than 0.8 nm. We further investigate the mode spacing of the RGB bands. At a pumping level above
As has been previously realized, the efficiency of upconversion lasing, especially that at higher lying states, is suppressed due to the depletion of energy related to the high nonradiative recombination loss of
Further evidence for stable white-light lasing behavior in the
Figure 9.Upconversion lasing characterization of
Figure 10.White-light lasing characterization of
This white-light microlaser is intrinsically different from the previous reports [9,12,13]. In conventional designs, the thresholds of RGB microlasers are quite different. As a result, the ratios between different colors change dramatically, strongly affecting the stability of the white-light laser. In contrast, the color coordinates of our white-light lasing roughly remain stable (i.e., above
3. CONCLUSION
In conclusion, we have demonstrated a high-
4. MATERIALS AND METHODS
Acknowledgment
Acknowledgment. The authors also acknowledge support from the Shenzhen Engineering Laboratory on Organic-Inorganic Perovskite Devices.
References
[1] L. Jin, X. Chen, Y. Wu, X. Ai, X. Yang, S. Xiao, Q. Song. Dual-wavelength switchable single-mode lasing from a lanthanide-doped resonator. Nat. Commun., 13, 1727(2022).
[2] B.-S. Moon, T. K. Lee, W. C. Jeon, S. K. Kwak, Y.-J. Kim, D.-H. Kim. Continuous-wave upconversion lasing with a sub-10 W cm−2 threshold enabled by atomic disorder in the host matrix. Nat. Commun., 12, 4437(2021).
[3] H. Xin, Y. Li, Y.-C. Liu, Y. Zhang, Y.-F. Xiao, B. Li. Optical forces: from fundamental to biological applications. Adv. Mater., 32, 2001994(2020).
[4] Y. Hu, L. Shao, S. Arnold, Y.-C. Liu, C.-Y. Ma, Y.-F. Xiao. Mode broadening induced by nanoparticles in an optical whispering-gallery microcavity. Phys. Rev. A, 90, 043847(2014).
[5] C. Zhang, C.-L. Zou, Y. Zhao, C.-H. Dong, C. Wei, H. Wang, Y. Liu, G.-C. Guo, J. Yao, Y. S. Zhao. Organic printed photonics: from microring lasers to integrated circuits. Sci. Adv., 1, e1500257(2015).
[6] C. Huang, C. Zhang, S. Xiao, Y. Wang, Y. Fan, Y. Liu, N. Zhang, G. Qu, H. Ji, J. Han. Ultrafast control of vortex microlasers. Science, 367, 1018-1021(2020).
[7] X.-R. Mao, Z.-K. Shao, H.-Y. Luan, S.-L. Wang, R.-M. Ma. Magic-angle lasers in nanostructured moiré superlattice. Nat. Nanotechnol., 16, 1099-1105(2021).
[8] F. Fan, S. Turkdogan, Z. Liu, D. Shelhammer, C. Z. Ning. A monolithic white laser. Nat. Nanotechnol., 10, 796-803(2015).
[9] D. Huang, Y. Xie, D. Lu, Z. Wang, J. Wang, H. Yu, H. Zhang. Demonstration of a white laser with V2C MXene-based quantum dots. Adv. Mater., 31, 1901117(2019).
[10] K. Ge, X. Shi, Z. Xu, L. Cui, T. Zhai. Full-color WGM lasing in nested microcavities. Nanoscale, 13, 10792-10797(2021).
[11] X. Shi, Y. Bian, J. Tong, D. Liu, Z. Wang. Chromaticity-tunable white random lasing based on a microfluidic channel. Opt. Express, 28, 13576-13585(2020).
[12] A. Adamow, A. Szukalski, L. Sznitko, L. Persano, D. Pisignano, A. Camposeo, J. Mysliwiec. Electrically controlled white laser emission through liquid crystal/polymer multiphases. Light Sci. Appl., 9, 19(2020).
[13] P. Guo, M. K. Hossain, X. Shen, H. Sun, W. Yang, C. Liu, C. Y. Ho, C. K. Kwok, S. Tsang, Y. Luo, J. C. Ho, K. M. Yu. Room-temperature red-green-blue whispering-gallery mode lasing and white-light emission from cesium lead halide perovskite (CsPbX3, X = Cl, Br, I) microstructures. Adv. Opt. Mater., 6, 1700993(2018).
[14] H.-L. Dai, C. Yin, Z.-Y. Xiao, Z.-Q. Cao, X.-F. Chen. White beam lasing from a hybrid microcavity with slab-capillary mode coupling. Phys. Rev. Appl., 11, 064055(2019).
[15] T. Wang, H. Yu, C. K. Siu, J. Qiu, X. Xu, S. F. Yu. White-light whispering-gallery-mode lasing from lanthanide-doped upconversion NaYF4 hexagonal microrods. ACS Photon., 4, 1539-1543(2017).
[16] G. Haider, H.-I. Lin, K. Yadav, K.-C. Shen, Y.-M. Liao, H.-W. Hu, P. K. Roy, K. P. Bera, K.-H. Lin, H.-M. Lee, Y.-T. Chen, F.-R. Chen, Y.-F. Chen. A highly-efficient single segment white random laser. ACS Nano, 12, 11847-11859(2018).
[17] L. Jin, Y. Wu, Y. Wang, S. Liu, Y. Zhang, Z. Li, X. Chen, W. Zhang, S. Xiao, Q. Song. Mass-manufactural lanthanide-based ultraviolet B microlasers. Adv. Mater., 31, 1807079(2019).
[18] X. Zhu, X. Yu, W. Gao, H. Liu, L. Nie, H. Guo, F. Zhao, S. Yu, T. Wang. Optical enhancement of nonstoichiometry-induced heterojunction in lanthanide doped double perovskite phosphors for WLEDs and scintillation applications. Chem. Eng. J., 442, 136235(2022).
[19] K. Zheng, Y. Liu, Z. Liu, Z. Chen, W. Qin. Color control and white upconversion luminescence of LaOF:Ln3+(Ln = Yb, Er, Tm) nanocrystals prepared by the sol-gel Pechini method. Dalton Trans., 42, 5159-5166(2013).
[20] C. Zhang, L. Yang, J. Zhao, B. Liu, M. Y. Han, Z. Zhang. White-light emission from an integrated upconversion nanostructure: toward multicolor displays modulated by laser power. Angew. Chem. Int. Ed., 127, 11693-11697(2015).
[21] P. Chen, Q. Li, S. Grindy, N. Holten-Andersen. White-light-emitting lanthanide metallogels with tunable luminescence and reversible stimuli-responsive properties. J. Am. Chem. Soc., 137, 11590-11593(2015).
[22] X. Chen, L. Jin, W. Kong, T. Sun, W. Zhang, X. Liu, J. Fan, S. F. Yu, F. Wang. Confining energy migration in upconversion nanoparticles towards deep ultraviolet lasing. Nat. Commun., 7, 10304(2016).
[23] T. Lu, L. Yang, R. V. A. Van Loon, A. Polman, K. J. Vahala. On-chip green silica upconversion microlaser. Opt. Lett., 34, 482-484(2009).
[24] S. Mehrabani, A. M. Armani. Blue upconversion laser based on thulium-doped silica microcavity. Opt. Lett., 38, 4346-4349(2013).
[25] T. J. Kippenberg, J. Kalkman, A. Polman, K. J. Vahala. Demonstration of an erbium-doped microdisk laser on a silicon chip. Phys. Rev. A, 74, 051802(2006).
[26] X.-F. Jiang, Y.-F. Xiao, C.-L. Zou, L. He, C.-H. Dong, B.-B. Li, Y. Li, F.-W. Sun, L. Yang, Q. Gong. Highly unidirectional emission and ultralow-threshold lasing from on-chip ultrahigh-
[27] L. Chang, X. Jiang, S. Hua, C. Yang, J. Wen, L. Jiang, G. Li, G. Wang, M. Xiao. Parity-time symmetry and variable optical isolation in active-passive-coupled microresonators. Nat. Photonics, 8, 524-529(2014).
[28] L. Yang, D. K. Armani, K. J. Vahala. Fiber-coupled erbium microlasers on a chip. Appl. Phys. Lett., 83, 825-826(2003).
[29] C. J. Bekker, C. G. Baker, W. P. Bowen. Optically tunable photoluminescence and up-conversion lasing on a chip. Phys. Rev. Appl., 15, 034022(2021).
[30] X. Chen, D. Peng, Q. Ju, F. Wang. Photon upconversion in core-shell nanoparticles. Chem. Soc. Rev., 44, 1318-1330(2015).
[31] G. Chen, H. Ågren, T. Y. Ohulchanskyy, P. N. Prasad. Light upconverting core–shell nanostructures: nanophotonic control for emerging applications. Chem. Soc. Rev., 44, 1680-1713(2015).
[32] F. Wang, R. Deng, J. Wang, Q. Wang, Y. Han, H. Zhu, X. Chen, X. Liu. Tuning upconversion through energy migration in core-shell nanoparticles. Nat. Mater., 10, 968-973(2011).
[33] H. Wen, H. Zhu, X. Chen, T. F. Hung, B. Wang, G. Zhu, S. F. Yu, F. Wang. Upconverting near-infrared light through energy management in core-shell-shell nanoparticles. Angew. Chem. Int. Ed., 52, 13419-13423(2013).
[34] T. Sun, B. Chen, Y. Guo, Q. Zhu, J. Zhao, Y. Li, X. Chen, Y. Wu, Y. Gao, L. Jin, S. T. Chu, F. Wang. Ultralarge anti-Stokes lasing through tandem upconversion. Nat. Commun., 13, 1032(2022).
[35] H. Zhu, X. Chen, L. M. Jin, Q. J. Wang, F. Wang, S. F. Yu. Amplified spontaneous emission and lasing from lanthanide-doped up-conversion nanocrystals. ACS Nano, 7, 11420-11426(2013).
[36] L. M. Jin, C. Xian, C. K. Siu, W. Feng, S. F. Yu. Enhancing multiphoton upconversion from NaYF4:Yb/Tm@NaYF4 core–shell nanoparticles via the use of laser cavity. ACS Nano, 11, 843-849(2017).
[37] S. Sivakumar, F. C. M. Van Veggel, P. S. May. Near-infrared (NIR) to red and green up-conversion emission from silica sol-gel thin films made with La0.45Yb0.50Er0.05F3 nanoparticles, hetero-looping-enhanced energy transfer (Hetero-LEET): a new up-conversion process. J. Am. Chem. Soc., 129, 620-625(2007).
[38] M. Yu, J. Lin, Z. Wang, J. Fu, S. Wang, H. J. Zhang, Y. C. Han. Fabrication, patterning, and optical properties of nanocrystalline YVO4:A (A = Eu3+, Dy3+, Sm3+, Er3+) phosphor films via sol-gel soft lithography. Chem. Mater., 14, 2224-2231(2002).
[39] G. Wang, Q. Peng, Y. Li. Upconversion luminescence of monodisperse CaF2:Yb3+/Er3+ nanocrystals. J. Am. Chem. Soc., 131, 14200-14201(2009).
[40] X. Chen, B. Zhang, X. Qian, J. Wang, L. Zheng, J. Hou, Y. Fang, L. Su. Upconversion color tenability and white light generation in Yb3+/Er3+/Tm3+ tri-doped CaF2 single crystals. Opt. Mater., 90, 40-45(2019).
[41] D. K. Armani, T. J. Kippenberg, S. M. Spillane, K. J. Vahala. Ultra-high-
[42] K. A. Knapper, K. D. Heylman, E. H. Horak, R. H. Goldsmith. Chip-scale fabrication of high-
[43] D. Korn, M. Lauermann, S. Koeber, P. Appel, L. Alloatti, R. Palmer, P. Dumon, W. Freude, J. Leuthold, C. Koos. Lasing in silicon–organic hybrid waveguides. Nat. Commun., 7, 10864(2016).
[44] Y. Wang, N. Zhang, Z. Jiang, L. Wang, Y. Xiao, W. Sun, N. Yi, S. Liu, X. Gu, S. Xiao, Q. Song. Chip-scale mass manufacturable high-
[45] L. Zhou, D. Chen, W. Luo, Y. Wang, Y. Yu, F. Liu. Transparent glass ceramic containing Er3+:CaF2 nano-crystals prepared by sol-gel method. Mater. Lett., 61, 3988-3990(2007).
[46] L. He, Ş. K. Özdemir, Y. Lan. Whispering gallery microcavity lasers. Laser Photonics Rev., 7, 60-82(2013).
[47] T. Wang, W. Lu, X. Xu, J. Qiu, S. F. Yu. Study of crystallization and coalescence of nanocrystals in amorphous glass at high temperature. Inorg. Chem., 58, 9500-9504(2019).
[48] B. Chen, D. Peng, X. Chen, X. Qiao, X. Fan, F. Wang. Establishing the structural integrity of core-shell nanoparticles against elemental migration using luminescent lanthanide probes. Angew. Chem. Int. Ed., 54, 12788-12790(2015).
[49] N. Zhang, Z. Gu, S. Liu, Y. Wang, S. Wang, Z. Duan, W. Sun, Y.-F. Xiao, S. Xiao, Q. Song. Far-field single nanoparticle detection and sizing. Optica, 4, 1151-1156(2017).
[50] J. Wang, R. Deng, M. A. Macdonald, B. Chen, J. Yuan, F. Wang, D. Chi, T. S. A. Hor, P. Zhang, G. Liu, Y. Han, X. Liu. Enhancing multiphoton upconversion through energy clustering at sublattice level. Nat. Mater., 13, 157-162(2014).
[51] C. Huang, W. Sun, S. Liu, S. Li, S. Wang, Y. Wang, N. Zhang, H. Fu, S. Xiao, Q. Song. Highly controllable lasing actions in lead halide perovskite-Si3N4 hybrid micro-resonators. Laser Photonics Rev., 13, 1800189(2019).
[52] C. Huang, W. Sun, Y. Fan, Y. Wang, Y. Gao, N. Zhang, K. Wang, S. Liu, S. Wang, S. Xiao, Q. Song. Formation of lead halide perovskite based plasmonic nanolasers and nanolaser arrays by tailoring the substrate. ACS Nano, 12, 3865-3874(2018).
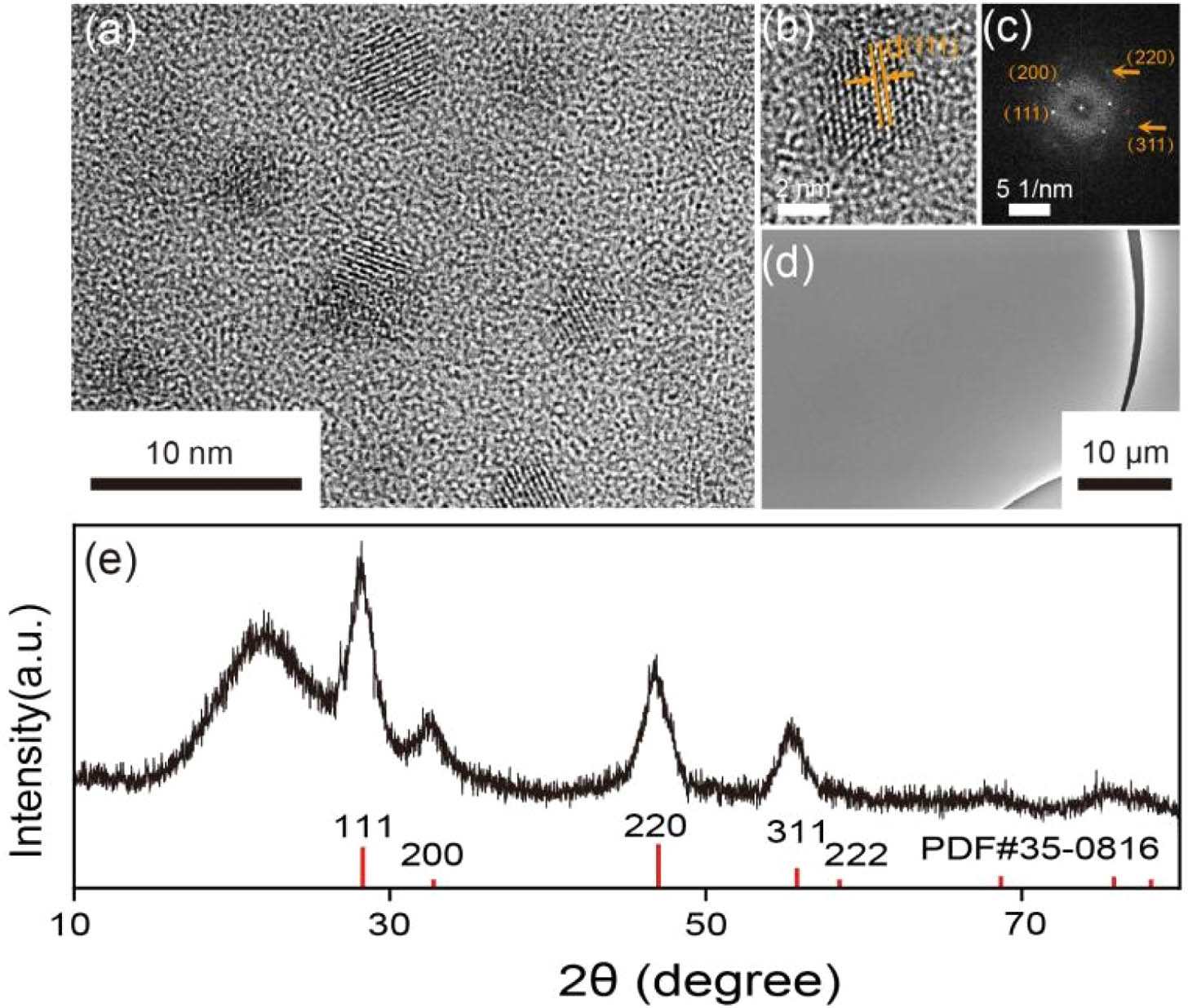
Set citation alerts for the article
Please enter your email address