
- Photonics Research
- Vol. 10, Issue 3, 731 (2022)
Abstract
1. Introduction
Optical whispering gallery mode (WGM) microresonator platforms featuring both high quality (
Cavity soliton combs typically coincide with an abrupt reduced power step. Due to thermal effects, such regimes usually require specific approaches to access [7,14]. Recently, cavity solitons forming a crystal-like structure, referred to as soliton crystals, were found [10,15–20]. Avoided mode crossing (AMX) induced modulation on an intracavity continuous-wave (CW) background is found to cause the ordering of multiple Kerr solitons [10]. These soliton crystal states appear with larger conversion efficiencies and are much easier to access compared to regular soliton states. Manual tuning of the pump frequency is enough to locate a self-thermally locked position into the soliton crystal step. AMX aided Kerr frequency comb generation in the normal dispersion regime has been reported in silicon nitride (
On the other hand, Kerr frequency comb generation also benefits from its host material platforms [25]. The property of a high refractive index leads to a better light confinement ability. Various high index materials have been exploited for Kerr comb generation. Moreover, host materials with other nonlinearities can also enrich comb performances through multi-nonlinearity [26]. For instance, Kerr and Pockel nonlinearities in a lithium niobate (
Sign up for Photonics Research TOC. Get the latest issue of Photonics Research delivered right to you!Sign up now
In this work, for the first time to our knowledge, we report the fabrication of YSZ optical microresonators with high-
2. EXPERIMENTAL METHODS
A. Fabrication of YSZ Microcavities
High-
Figure 1.(a) Illustrated fabrication method of YSZ microdisk resonator. (b) Left to right: side view photo of a YSZ microresonator mounted on an aluminum post; zoomed-in photo and top view of the microdisk showing a measured major radius
Also shown is the field distribution of several transverse mode families of WGMs. Besides the polarization of transverse electric (TE) and transverse magnetic (TM) modes, WGMs are characterized by three mode orders determining their field distributions. The azimuthal order
B. Experimental Setup
As the YSZ crystal features a refractive index of 2.11 at the telecom wavelength of 1550 nm, the rutile (
Figure 2.(a) Schematic of the experimental setup. FC, fiber coupler; SF, single mode fiber; EDFA, erbium doped fiber amplifier; VOA, variable optical attenuator; FPC, fiber polarization controller; FR: fiber ring etalon; FOC, fiber optical circulator; L, optical lens; PD, photodetector; MF, multimode fiber; FG, function generator; OSC, digital oscilloscope; OSA, optical spectrum analyzer. (b) Top: photo of the YSZ microresonator and
3. RESULTS AND DISCUSSION
A. Q Factors at the Telecommunication Wavelength
The evanescent coupling gap between the microresonator and the prism was controlled through a piezo-actuator. The photo of the coupled resonator is shown in Fig. 2(b) (top). Also presented in this figure is the laser-scanned transmission spectrum across an under-coupled WGM of the YSZ microresonator. A Lorentzian fit of the resonance peak gives a linewidth of 2.4 MHz around 1550 nm, indicating an intrinsic
However, it was found that the intrinsic
B. Mode Crossing
It has been shown that the generation of soliton crystal combs is linked to mode crossing phenomena in microcavities [10]. Here, we carried out laser-scanned spectroscopy on cavity A featuring a few modes within one FSR to reveal mode crossing effects. Figure 3(a) shows the spectroscopy result over a 17 nm spectral window. The bottom curve is the simultaneously recorded fiber etalon signals as frequency markers. It is found that the free spectral range of the cavity around 1550 nm is about 0.6 nm through both optical spectrum and transmission spectrum analysis. One clearly sees that only two mode families feature the best mode contrast over 20% within one FSR. Zoomed-in spectra as shown in the inset of Fig. 3(a) give two examples of the mode spacing between these two transverse modes (A and B). We plot the absolute values of mode spacing between modes A and B families as a function of the center wavelengths between them in Fig. 3(b). The mode crossing phenomenon is clearly visible around 1553.4 nm where the mode spacing reaches its minimum. Examples of Kerr frequency comb spectra when pumping mode A families are presented as insets. We observe the pinning phenomenon of the first comb line around the crossing regime. Abrupt transmission steps appear to be coincident with the comb generation, in agreement with reported soliton crystal generation [15]. For the pump mode in the mode crossing regime where the spacing between modes A and B reaches the minimum, we find the generation of a symmetric Kerr comb.
Figure 3.Mode crossing in the YSZ microresonator. (a) Laser-scanned transmission spectrum covering a 30-FSR spectral range of the YSZ microresonator (cavity A) with fiber etalon signals simultaneously captured for wavelength or frequency range calibration. Red crosses: mode A family. Blue circles: mode B family. FSR, FSR of WGMs;
Through observing the spacing distribution of each mode family in Fig. 3, it is still difficult to determine the mode coupling conditions. We thereby further derive the FSR values of modes A and B families. For clarity, we first plot relative frequency positions of both modes A and B families in Fig. 4(a). A constant increment of one FSR value
Figure 4.Mode coupling in the YSZ microcavity. (a) Relative frequency position of modes A and B families showing a crossing position near 1554 nm. (b) FSR values of modes A and B families with the observed weak mode coupling induced disturbance. Solid lines: calculated analytical estimated data.
To theoretically investigate the total dispersion profile of this cavity, we calculated the eigenfrequencies by using the analytical approximation of WGMs in a toroid [44,45]. We use a major radius of 300.24 μm and a minor radius of 118.18 μm for the calculation. The Sellmeier equation of YSZ is utilized to take into account the material dispersion. By solving the formulation, we find that the estimated eigenfrequencies of
In general, anomalous dispersion refers to decreased FSR values as wavelengths increase and vice versa [39]. When second order dispersion
1. Generation of Soliton and Crystals in the Few-Mode Cavity
YSZ cavity A was fabricated such that only the sharp rim of the disk was selectively fine polished. As a result, many high-order transverse WGMs encounter higher scattering losses when their mode fields reach the rough surface. Furthermore, the prism coupling setup can be used to excite mainly low-order modes with an optimized incident angle of the input beam. Therefore, we were able to observe the few-mode spectrum in such a cavity as shown in Fig. 3. We refer to this cavity as the few-mode cavity.
The generation of Kerr frequency combs relies strongly on many parameters including pump power, detuning frequency of the pump laser from the mode, and the dispersion profile. Versatile comb regimes can be excited depending on parameters such as an MI comb, chaotic comb, breathing solitons, single solitons, and soliton crystals [10]. The pump power should not be too much above threshold for accessing phase-locked solitons. The threshold of a Kerr frequency comb can be estimated by [46]
We then use
Due to the presence of Rayleigh scattering in the high-
Figure 5 shows the observation of a single soliton frequency comb transition in YSZ cavity A when pumping mode A at 1554.7 nm. The coupling condition was under-coupled. The appearance of the soliton step in the transmission spectrum is highlighted with a green background in Fig. 5(a). We find that this step accompanies the observation of Kerr combs in the normal dispersion regime, similar to that of the reported mode crossing induced soliton crystals [15]. The self-thermal locking regime can be easily accessed when pump mode A is on the blue side of mode B. In this experiment, we stopped laser scanning and manually located the thermal locking of the pump frequency into mode A. We observed a comb spectrum clearly disrupted by the mode crossing as shown in Fig. 5(b) (left). The first comb line appears to be pinned at the mode around 1554.1 nm, while the comb line power encountered a sudden drop at the mode around 1553.5 nm. It is consistent with the reported pinning effect and the envelope disruption of the mode crossing in the Kerr comb spectrum [21,43]. However, when we further redshifted the wavelength of the pump laser in regime II, a stable single FSR spaced soliton comb was then formed as shown in Fig. 5(b) (right). The envelope of the comb fits well with a
Figure 5.Soliton and soliton crystal frequency comb generation pumped at mode A family. (a) Pump scanned transmission spectrum around 1554.7 nm over about 6 GHz spectral range showing a soliton step coincident with the increased feedback power regime. (b) Comb spectra obtained with the pump thermally locked to mode A. Dashed line:
We further chose to pump other adjacent mode A families and observed similar transmission steps in the forward direction, while an abrupt increased signal power step appeared in the backward direction. Using self-thermal locking [48], we obtained the generation of soliton crystal combs with different comb line spacings. Figure 5(c) shows examples of such spectra with 2-FSR, 3-FSR, 9-FSR, and 10-FSR spacings when the pump modes are around 1555.3 nm, 1555.9 nm, 1559.5 nm, and 1560.1 nm, respectively. It is found that the pinned first comb lines all appear around 1554.1 nm. The power of this comb line is found to be stronger than others. In particular, we observed wider comb spectra with line spacing from 3-FSR to 6-FSR with dispersive-wave-like tails as shown in Fig. 5(d), which could result from the dispersive wave induced by other mode interactions [43].
Furthermore, we studied the evolution of the soliton crystal combs in such a cavity as shown in Fig. 6. The pump laser frequency was first scanned across mode A around 1555.8 nm, and the coupling gap was optimized for comb generation. We then stopped the laser ramping, manually tuned the laser wavelength into the resonance, and recorded the corresponding comb spectra. Figures 6(a)–6(c) present comb spectra in three different detuning regimes marked in the transmission spectrum in the inset of Fig. 6(d). As the wavelength was redshifted and self-thermally locked to the resonance, the primary comb with 3-FSR spacing, the breathing comb with 1-FSR spacing, and the soliton crystal comb with 3-FSR spacing were observed, respectively. We used the fast Fourier transform (FFT) function of the oscilloscope (
Figure 6.Evolution of soliton crystal comb generation during pump laser scanning. (a)–(c) Typical optical comb spectra. (d)–(f) Corresponding RF spectra obtained by FFT in the oscilloscope. Inset in (d): transmission spectrum in cavity A when the laser wavelength is scanned across mode A around 1555.8 nm. Inset in (e): corresponding temporal trace in the oscilloscope.
2. Kerr Combs in an Over-Mode Microresonator
In addition, we also investigate the comb generation with another YSZ microresonator (cavity B) with a major radius of about 456 μm. This resonator supports rich transverse high-
Figure 7.Kerr optical frequency comb generation in a YSZ microresonator with a radius of about 456 μm (cavity B). (a) Transmission spectrum spanning a spectral window of 11.9 GHz showing two adjacent WGMs with soliton crystal steps. Inset: photo of the microcavity coupled with the rutile prism. The appearance of the transmission step of mode A is recorded (see
When the pump laser was thermally locked to the transmission step of mode A in cavity B, we found that Kerr combs with very different frequency spacings could be generated depending on the detuning of the pump. For instance, the Kerr comb with 4-FSR or 0.2 THz frequency spacing transited into the comb with 30-FSR or 1.48 THz frequency spacing when the pump wavelength was redshifted. Further redshifting the pump wavelength, a very asymmetric comb with 3-FSR spacing was obtained as shown in Fig. 7(b). For pump mode B, we observed a relatively weaker feedback signal. However, it should be mentioned that the feedback strength of a Kerr comb in the cavity does not rely merely on comb power, since it is also determined by Rayleigh scattering strength. When pumping into this mode, we found less complicated comb transitions. A comb with 1.24 THz spacing or 25-FSR’ was observed as shown in Fig. 7(c).
4. CONCLUSION
To the best of our knowledge, we have demonstrated the fabrication of the first high-
Performances of Various Kerr Microcombs on High Refractive Index (
Material | Refractive Index | FSR (GHz) | Mode Area ( | Loaded | Lowest Threshold (mW) | |
---|---|---|---|---|---|---|
2.0 | 200 | 0.33 [ | ||||
AlN | 2.1 | 364 | 55 [ | |||
2.21 | 200 | 4.2 [ | ||||
Diamond | 2.4 | 925 | 20 [ | |||
4H silicon carbide | 2.6 | 260 | 10 [ | |||
Gallium phosphide | 3.1 | 250 | 3 [ | |||
AlGaAs | 3.3 | 1000 | 0.036 [ | |||
Si (pumped at 2.6 μm) | 3.5 | 127 | 3 [ | |||
YSZ (this work) | 2.1 | 74.5 | 1.9 |
References
[1] D. V. Strekalov, C. Marquardt, A. B. Matsko, H. G. Schwefel, G. Leuchs. Nonlinear and quantum optics with whispering gallery resonators. J. Opt., 18, 123002(2016).
[2] I. Breunig. Three-wave mixing in whispering gallery resonators. Laser Photon. Rev., 10, 569-587(2016).
[3] L. Ge, L. Feng, H. G. Schwefel. Optical microcavities: new understandings and developments. Photon. Res., 5, OM1-OM3(2017).
[4] G. Lin, A. Coillet, Y. K. Chembo. Nonlinear photonics with high-
[5] G. Lin, Y. K. Chembo. Monolithic total internal reflection resonators for applications in photonics. Opt. Mater. X, 2, 100017(2019).
[6] A. Pasquazi, M. Peccianti, L. Razzari, D. J. Moss, S. Coen, M. Erkintalo, Y. K. Chembo, T. Hansson, S. Wabnitz, P. Del’Haye, X. Xue, A. M. Weiner, R. Morandotti. Micro-combs: a novel generation of optical sources. Phys. Rep., 729, 1-81(2018).
[7] T. J. Kippenberg, A. L. Gaeta, M. Lipson, M. L. Gorodetsky. Dissipative Kerr solitons in optical microresonators. Science, 361, eaan8083(2018).
[8] A. L. Gaeta, M. Lipson, T. J. Kippenberg. Photonic-chip-based frequency combs. Nat. Photonics, 13, 158-169(2019).
[9] C. Godey, I. V. Balakireva, A. Coillet, Y. K. Chembo. Stability analysis of the spatiotemporal Lugiato-Lefever model for Kerr optical frequency combs in the anomalous and normal dispersion regimes. Phys. Rev. A, 89, 063814(2014).
[10] M. Karpov, M. H. Pfeiffer, H. Guo, W. Weng, J. Liu, T. J. Kippenberg. Dynamics of soliton crystals in optical microresonators. Nat. Phys., 15, 1071-1077(2019).
[11] S. Wan, R. Niu, Z.-Y. Wang, J.-L. Peng, M. Li, J. Li, G.-C. Guo, C.-L. Zou, C.-H. Dong. Frequency stabilization and tuning of breathing solitons in Si3N4 microresonators. Photon. Res., 8, 1342-1349(2020).
[12] H. Weng, J. Liu, A. A. Afridi, J. Li, J. Dai, X. Ma, Y. Zhang, Q. Lu, J. F. Donegan, W. Guo. Directly accessing octave-spanning dissipative Kerr soliton frequency combs in an AlN microresonator. Photon. Res., 9, 1351-1357(2021).
[13] X. Wang, P. Xie, W. Wang, Y. Wang, Z. Lu, L. Wang, S. T. Chu, B. E. Little, W. Zhao, W. Zhang. Program-controlled single soliton microcomb source. Photon. Res., 9, 66-72(2021).
[14] W. Wang, L. Wang, W. Zhang. “Advances in soliton microcomb generation. Adv. Photon., 2, 034001(2020).
[15] D. C. Cole, E. S. Lamb, P. Del’Haye, S. A. Diddams, S. B. Papp. Soliton crystals in Kerr resonators. Nat. Photonics, 11, 671-676(2017).
[16] W. Wang, Z. Lu, W. Zhang, S. T. Chu, B. E. Little, L. Wang, X. Xie, M. Liu, Q. Yang, L. Wang, J. Zhao, G. Wang, Q. Sun, Y. Liu, Y. Wang, W. Zhao. Robust soliton crystals in a thermally controlled microresonator. Opt. Lett., 43, 2002-2005(2018).
[17] Y. He, J. Ling, M. Li, Q. Lin. Perfect soliton crystals on demand. Laser Photon. Rev., 14, 1900339(2020).
[18] Z. Lu, H.-J. Chen, W. Wang, L. Yao, Y. Wang, Y. Yu, B. Little, S. Chu, Q. Gong, W. Zhao, X. Yi, Y.-F. Xiao, W. Zhang. Synthesized soliton crystals. Nat. Commun., 12, 3179(2021).
[19] H. Weng, A. A. Afridi, J. Liu, J. Li, J. Dai, X. Ma, Y. Zhang, Q. Lu, W. Guo, J. F. Donegan. Near-octave-spanning breathing soliton crystal in an AlN microresonator. Opt. Lett., 46, 3436-3439(2021).
[20] T. Huang, J. Pan, Z. Cheng, G. Xu, Z. Wu, T. Du, S. Zeng, P. P. Shum. Nonlinear-mode-coupling-induced soliton crystal dynamics in optical microresonators. Phys. Rev. A, 103, 023502(2021).
[21] Y. Liu, Y. Xuan, X. Xue, P.-H. Wang, S. Chen, A. J. Metcalf, J. Wang, D. E. Leaird, M. Qi, A. M. Weiner. Investigation of mode coupling in normal-dispersion silicon nitride microresonators for Kerr frequency comb generation. Optica, 1, 137-144(2014).
[22] X. Xue, Y. Xuan, P.-H. Wang, Y. Liu, D. E. Leaird, M. Qi, A. M. Weiner. Normal-dispersion microcombs enabled by controllable mode interactions. Laser Photon. Rev., 9, L23-L28(2015).
[23] M. Tan, X. Xu, A. Boes, B. Corcoran, J. Wu, T. G. Nguyen, S. T. Chu, B. E. Little, R. Morandotti, A. Mitchell, D. J. Moss. Photonic RF arbitrary waveform generator based on a soliton crystal micro-comb source. J. Lightwave Technol., 38, 6221-6226(2020).
[24] X. Xu, M. Tan, J. Wu, A. Boes, T. G. Nguyen, S. T. Chu, B. E. Little, R. Morandotti, A. Mitchell, D. J. Moss. Broadband photonic RF channelizer with 92 channels based on a soliton crystal microcomb. J. Lightwave Technol., 38, 5116-5121(2020).
[25] A. Kovach, D. Chen, J. He, H. Choi, A. H. Dogan, M. Ghasemkhani, H. Taheri, A. M. Armani. Emerging material systems for integrated optical Kerr frequency combs. Adv. Opt. Photon., 12, 135-222(2020).
[26] G. Lin, Q. Song. Kerr frequency comb interaction with Raman, Brillouin, and second order nonlinear effects. Laser Photon. Rev., 16, 2100184(2022).
[27] Y. He, Q.-F. Yang, J. Ling, R. Luo, H. Liang, M. Li, B. Shen, H. Wang, K. Vahala, Q. Lin. Self-starting bi-chromatic LiNBO3 soliton microcomb. Optica, 6, 1138-1144(2019).
[28] G. Marcaud, S. Serna, K. Panaghiotis, C. Alonso-Ramos, X. L. Roux, M. Berciano, T. Maroutian, G. Agnus, P. Aubert, A. Jollivet, A. Ruiz-Caridad, L. Largeau, N. Isac, E. Cassan, S. Matzen, N. Dubreuil, M. Rérat, P. Lecoeur, L. Vivien. Third-order nonlinear optical susceptibility of crystalline oxide yttria-stabilized zirconia. Photon. Res., 8, 110-120(2020).
[29] K. K. Gopalan, D. Rodrigo, B. Paulillo, K. K. Soni, V. Pruneri. Ultrathin yttria-stabilized zirconia as a flexible and stable substrate for infrared nano-optics. Adv. Opt. Mater., 7, 1800966(2019).
[30] G. Marcaud, S. Matzen, C. Alonso-Ramos, X. Le Roux, M. Berciano, T. Maroutian, G. Agnus, P. Aubert, L. Largeau, V. Pillard, S. Serna, D. Benedikovic, C. Pendenque, E. Cassan, D. Marris-Morini, P. Lecoeur, L. Vivien. High-quality crystalline yttria-stabilized-zirconia thin layer for photonic applications. Phys. Rev. Mater., 2, 035202(2018).
[31] X. D. Wu, S. R. Foltyn, P. N. Arendt, W. R. Blumenthal, I. H. Campbell, J. D. Cotton, J. Y. Coulter, W. L. Hults, M. P. Maley, H. F. Safar, J. L. Smith. Properties of YBa2Cu3O7-δ thick films on flexible buffered metallic substrates. Appl. Phys. Lett., 67, 2397-2399(1995).
[32] X. Hong, S. Xu, X. Wang, D. Wang, S. Li, B. A. Goodman, W. Deng. Growth, structure and optical spectroscopic properties of dysprosia-doped cubic yttria stabilized zirconia (YSZ) single crystals. J. Lumin., 231, 117766(2021).
[33] W. Liang, A. A. Savchenkov, V. S. Ilchenko, D. Eliyahu, D. Seidel, A. B. Matsko, L. Maleki. Generation of a coherent near-infrared Kerr frequency comb in a monolithic microresonator with normal GVD. Opt. Lett., 39, 2920-2923(2014).
[34] G. Lin, S. Diallo, R. Henriet, M. Jacquot, Y. K. Chembo. Barium fluoride whispering-gallery-mode disk-resonator with one billion quality-factor. Opt. Lett., 39, 6009-6012(2014).
[35] S. Fujii, Y. Hayama, K. Imamura, H. Kumazaki, Y. Kakinuma, T. Tanabe. All-precision-machining fabrication of ultrahigh-
[36] A. Danilin, G. Slinkov, V. Lobanov, K. Min’kov, I. Bilenko. Magneto-optical effects in a high-
[37] G. Lin, B. Qian, F. Oručević, Y. Candela, J.-B. Jager, Z. Cai, V. Lefèvre-Seguin, J. Hare. Excitation mapping of whispering gallery modes in silica microcavities. Opt. Lett., 35, 583-585(2010).
[38] G. Lin, Y. K. Chembo. On the dispersion management of fluorite whispering-gallery mode resonators for Kerr optical frequency comb generation in the telecom and mid-infrared range. Opt. Express, 23, 1594-1604(2015).
[39] S. Fujii, T. Tanabe. Dispersion engineering and measurement of whispering gallery mode microresonator for Kerr frequency comb generation. Nanophotonics, 9, 1087-1104(2020).
[40] X. Zhang, G. Lin, T. Sun, Q. Song, G. Xiao, H. Luo. Dispersion engineering and measurement in crystalline microresonators using a fiber ring etalon. Photon. Res., 9, 2222-2229(2021).
[41] G. Lin, J. Fürst, D. V. Strekalov, I. S. Grudinin, N. Yu. High-
[42] J. Li, H. Lee, K. Y. Yang, K. J. Vahala. Sideband spectroscopy and dispersion measurement in microcavities. Opt. Express, 20, 26337-26344(2012).
[43] Q.-F. Yang, X. Yi, K. Y. Yang, K. J. Vahala. Spatial-mode-interaction-induced dispersive waves and their active tuning in microresonators. Optica, 3, 1132-1135(2016).
[44] Y. A. Demchenko, M. L. Gorodetsky. Analytical estimates of eigenfrequencies, dispersion, and field distribution in whispering gallery resonators. J. Opt. Soc. Am. B, 30, 3056-3063(2013).
[45] I. Breunig, B. Sturman, F. Sedlmeir, H. G. L. Schwefel, K. Buse. Whispering gallery modes at the rim of an axisymmetric optical resonator: analytical versus numerical description and comparison with experiment. Opt. Express, 21, 30683-30692(2013).
[46] L. Chang, W. Xie, H. Shu, Q.-F. Yang, B. Shen, A. Boes, J. D. Peters, W. Jin, C. Xiang, S. Liu, G. Moille, S.-P. Yu, X. Wang, K. Srinivasan, S. B. Papp, K. Vahala, J. E. Bowers. Ultra-efficient frequency comb generation in AlGaAs-on-insulator microresonators. Nat. Commun., 11, 1331(2020).
[47] G. Lin, Y. K. Chembo. Phase-locking transition in Raman combs generated with whispering gallery mode resonators. Opt. Lett., 41, 3718-3721(2016).
[48] G. Lin, Y. Candela, O. Tillement, Z. Cai, V. Lefèvre-Seguin, J. Hare. Thermal bistability-based method for real-time optimization of ultralow-threshold whispering gallery mode microlasers. Opt. Lett., 37, 5193-5195(2012).
[49] C. Bao, Y. Xuan, C. Wang, A. Fülöp, D. E. Leaird, V. Torres-Company, M. Qi, A. M. Weiner. Observation of breathing dark pulses in normal dispersion optical microresonators. Phys. Rev. Lett., 121, 257401(2018).
[50] A. A. Savchenkov, A. B. Matsko, W. Liang, V. S. Ilchenko, D. Seidel, L. Maleki. Kerr frequency comb generation in overmoded resonators. Opt. Express, 20, 27290-27298(2012).
[51] X. Ji, F. A. Barbosa, S. P. Roberts, A. Dutt, J. Cardenas, Y. Okawachi, A. Bryant, A. L. Gaeta, M. Lipson. Ultra-low-loss on-chip resonators with sub-milliwatt parametric oscillation threshold. Optica, 4, 619-624(2017).
[52] H. Weng, J. Liu, A. A. Afridi, J. Li, J. Dai, X. Ma, Y. Zhang, Q. Lu, J. F. Donegan, W. Guo. Octave-spanning Kerr frequency comb generation with stimulated Raman scattering in an AlN microresonator. Opt. Lett., 46, 540-543(2021).
[53] B. Hausmann, I. Bulu, V. Venkataraman, P. Deotare, M. Lončar. Diamond nonlinear photonics. Nat. Photonics, 8, 369-374(2014).
[54] C. Wang, Z. Fang, A. Yi, B. Yang, Z. Wang, L. Zhou, C. Shen, Y. Zhu, Y. Zhou, R. Bao, Z. Li, Y. Chen, K. Huang, J. Zhang, Y. Cheng, X. Ou. High-
[55] D. J. Wilson, K. Schneider, S. Hönl, M. Anderson, Y. Baumgartner, L. Czornomaz, T. J. Kippenberg, P. Seidler. Integrated gallium phosphide nonlinear photonics. Nat. Photonics, 14, 57-62(2020).
[56] M. Pu, L. Ottaviano, E. Semenova, K. Yvind. Efficient frequency comb generation in AlGaAs-on-insulator. Optica, 3, 823-826(2016).
[57] A. G. Griffith, R. K. W. Lau, J. Cardenas, Y. Okawachi, A. Mohanty, R. Fain, Y. H. D. Lee, M. Yu, C. T. Phare, C. B. Poitras, A. L. Gaeta, M. Lipson. Silicon-chip mid-infrared frequency comb generation. Nat. Commun., 6, 6299(2015).
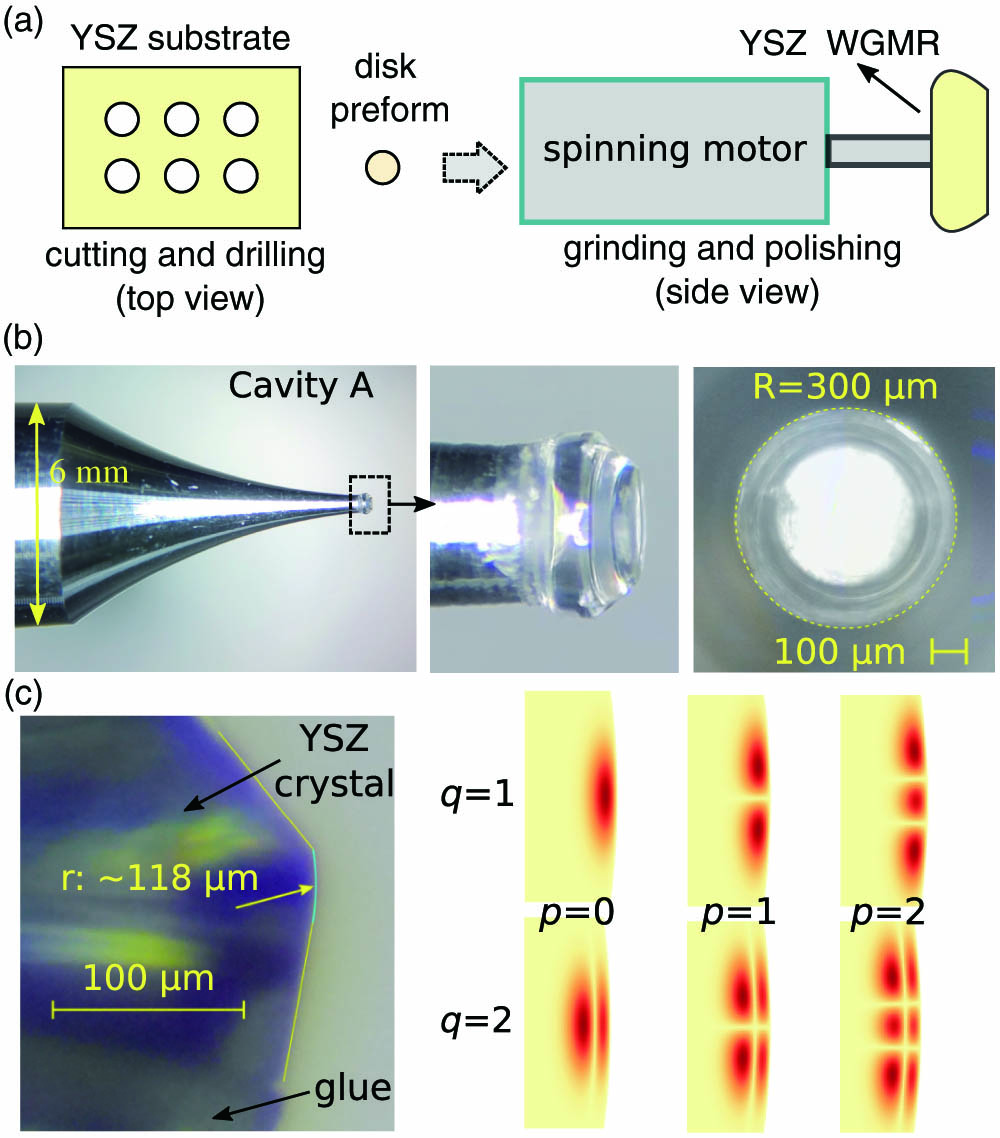
Set citation alerts for the article
Please enter your email address