
- Chinese Optics Letters
- Vol. 20, Issue 6, 062501 (2022)
Abstract
1. Introduction
Since the development of weak optical signal recognition technology, the research community has made many important discoveries in optical information fields, such as optical communication, artificial intelligence, and Internet of Things[
At present, commercial InGaAs/InP APD in the Geiger mode has been widely used in weak light detection with a wavelength of 1550 nm[
2. Device Design and Fabrication
The APDs were fabricated in a standard Si-photonics platform. The structure diagram of the device is shown in Fig. 1. Phosphorus and boron implantation in the 220 nm top Si substrate was performed to form N and P-type regions. The heavily doped N++- and P++-regions are used to form ohmic contact with Al electrodes. The P region with doping concentration of
Figure 1.Structure diagram of the lateral SACM Ge-on-Si APD.
As depicted in Fig. 2, When the APD operates at a low reverse bias, the P region located in the middle of two intrinsic regions is not depleted, resulting in the electric field unable to extend to the I1 region effectively, and the energy band of electrons in the I1 region is lower than that in the P-type region. Therefore, the electrons in the I1 region hardly overcome the barrier and cannot transit to the I2 region successfully, which inhibits the dark current effectively. With a high bias applied, the electric field in the I2 region is high enough to induce the avalanche of the device. At the same time, the P region is fully depleted, and the electric field in the I1 region is also improved, resulting in the energy band in the P region being lower than the one in the I1 region, and the free electrons effectively can move to the avalanche region for multiplication. At the same time, the electric field in the I1 region is much lower than
Figure 2.(a) Distribution of valence and conduction bands in the I1 region at -2 V and -9 V (Vex = 20%Vbr). (b) The electric field distribution in the Si substrate at -2 V and -9 V.
3. Device Performance
To study the response performance of the device, a 1550 nm CW light signal was guided to an optical grating, and the insertion loss is about
Figure 3(a) shows the I-V curves of the device at the optical power in the range of
Figure 3.(a) Photo-dark current of the SACM APD. (b) Avalanche probability simulation of the SACM APD.
The
Compared with the existing Ge-on-Si APDs with a wide avalanche region, the avalanche region of the device is only about 0.4 µm, and there is a risk that the APD will be burned down if the excess bias (
Figure 4.(a) Setup of the weak light signal detection experiment. The red lines are the optical paths, and the black ones represent the electrical paths. (b) Diagram of the device under test (DUT).
The DCR of the APD in Geiger mode was studied first. To suppress the after pulsing noise of the device, we used a series of pulses with repetition frequency in the range of 2 kHz to 20 kHz and pulse width
Figure 5.(a) DCR of the detection system versus excess bias at 300 K. (b) DCR of the detection system versus repetition rate of gate pulse with 20% excess bias applied.
Next, the optical response performance of the lateral SACM Ge-on-Si APD system for 1550 nm weak coherent pulse was investigated. Considering that the sensitivity of the APD is limited at 300 K, our study focused on the detection efficiency
Figure 6.(a) Detection efficiency η of the detection system versus excess bias at 300 K. (b) NEP of the detection system versus excess bias at 300 K.
It has been reported that a balanced detector structure formed by two identical APDs in parallel can improve the sensitivity of detection system[
Finally, we investigated the time jitter of the lateral SACM APD in Geiger mode. Because the accuracy of the TDC we used is only 50 ps, which is far larger than those used in other reports, time jitter of the detection system cannot be calculated accurately. Through Gaussian fitting of the time count histogram data recorded by TDC, the FWHM of histogram in Fig. 7 is estimated to be about 585 ps. The corresponding system jitter calculated by Eq. (4) is 248.4 ps, which includes the jitter of the laser, the jitter of the RF amplifier, and the jitter of the Ge-on-Si APD[
Figure 7.Histograms of the DUT at 20% excess bias.
4. Conclusion
To address the problems of the high DCR of the Ge-on-Si APD at 1550 nm and room temperature, this work proposes a Ge-on-Si APD with a lateral SACM structure. By optimizing the concentrations and widths of the doped regions, the electric field in the Ge–Si contact surface of the device is effectively suppressed, and the primary dark current of the APD in linear mode is only about
Reference | Temperature (K) | DCR (cps) | Wavelength (nm) | ||
---|---|---|---|---|---|
[ | 80 | 1310 | 0.1 | 5.27 | |
[ | 78 | 1310 | – | 31 | |
[ | 125 | 1310 | 0.01 | 29.4 | |
This Work | 300 | 1550 | 1 | 7.8 |
Table 1. Detection Performance of Ge-on-Si APD in Geiger Mode
References
[1] P. A. Govind. Fiber-Optic Communication Systems(2002).
[2] A. N. Otte, J. Barral, B. Dolgoshein, J. Hose, M. Teshima. New results from a test of silicon photomultiplier as readout for PET. Nuclear Science Symposium/Medical Imaging Conference, 3738(2004).
[3] S. Cova, M. Ghioni, I. Rech, F. Zappa. Evolution and prospects for single-photon avalanche diodes and quenching circuits. J. Mod. Opt., 51, 1267(2003).
[4] H. Chen, P. Verheyen, P. D. Heyn, G. Lepage, J. V. Campenhout. −1 V bias 67 GHz bandwidth Si-contacted germanium waveguide p-i-n photodetector for optical links at 56 Gbps and beyond. Opt. Express, 24, 4622(2016).
[5] H. -T. Chen, P. Verheyen, M. Rakowski, P. D. Heyn, G. Lepage, J. D. Coster, P. Absil, G. Roelkens, J. V. Campenhout. High-responsivity low-voltage 28-Gb/s Ge p-i-n photodetector with silicon contacts. J. Lightwave Technol., 33, 820(2015).
[6] Y.-X. Li, X.-B. Liu, X.-T. Li, G.-Q. Lo, J.-F. Song. Surface illuminated interdigitated Ge-on-Si photodetector with high responsivity. Opt. Express, 29, 16346(2021).
[7] N. Duan, T.-Y. Liow, A. E.-J. Lim, L. Ding, G.-Q. Lo. 310 GHz gain-bandwidth product Ge/Si avalanche photodetector for 1550 nm light detection. Opt. Express, 20,, 11031(2012).
[8] X. -G. Zeng, Z. -H. Huang, B. -H. Wang, M. Fiorentino, R. G. Beausoleil. Silicon–germanium avalanche photodiodes with direct control of electric field in charge multiplication region. Optica, 6, 772(2019).
[9] Z.-H. Huang, C. Li, D. Liang, K.-Z Yu, S. Palermo, R. G. Beausoleil. 25 Gbps low-voltage waveguide Si–Ge avalanche photodiode. Optica, 3, 793(2016).
[10] N. J. D. Martinez, C. T. Derose, R. W. Brock, A. L. Starbuck, A. T. Pomerene, A. L. Lentine, D. C. Trotter, P. S. Davids. High performance waveguide-coupled Ge-on-Si linear mode avalanche photodiodes. Opt. Express, 24, 19072(2016).
[11] S. A. Srinivasan, M. Berciano, P. S. Lardenois, M. Pantouvaki, J. Van Campenhout. 27 GHz silicon-contacted waveguide-coupled Ge/Si avalanche photodiode. J. Lightwave Technol., 38, 3044(2020).
[12] Y. Yuan, Z.-H. Huang, X.-G. Zeng, D. Liang, W. V. Sorin, M. Fiorentino, R. G. Beausoleil. High responsivity Si-Ge waveguide avalanche photodiodes enhanced by loop reflector. IEEE J. Sel. Top. Quantum Electron., 28, 3800508(2022).
[13] D. Benedikovic, L. Virot, G. Aubin, J. M. Hartmann, F. Amar, X. L. Roux, C. A. Ramos, B. Szelag, L. Vivien. Silicon-germanium avalanche receivers with fJ/bit energy consumption. IEEE J. Sel. Top. Quantum Electron., 28, 3802508(2022).
[14] Y. Li, X. Luo, G. Liang, G. Q. Lo. Demonstration of Ge/Si avalanche photodetector arrays for Lidar application. Optical Fiber Communication Conference, 1(2019).
[15] D. R. Gozzard, L. E. Roberts, J. T. Spollard, P. G. Sibley, D. A. Shaddock. Fast beam steering with an optical phased array. Opt. Lett., 45, 3793(2020).
[16] D.-W. Zhuang, L.-X. Zhang, X.-C. Han, Y.-X. Li, F.-L. Gao, J. -F. Song. Omnidirectional beam steering using aperiodic optical phased array with high error margin. Opt. Express, 26, 19154(2018).
[17] L.-X. Zhang, Y.-Z. Li, Y. Hu, Y.-B. Wang, M. Tao, B.-S. Chen, Q.-X. Na, Y.-X. Li, Z.-H. Zhi, X.-B. Liu, X.-Y. Li, F.-L. Gao, X.-S. Luo, G.-Q. Lo, J.-F. Song. Investigation and demonstration of a high-power handling and large-range steering optical phased array chip. Opt. Express, 29, 29755(2021).
[18] L.-X. Zhang, Y.-Z. Li, M. Tao, Y.-B. Wang, Y. Hu, B.-S. Chen, Y.-X. Li, G.-Q. Lo, J.-F. Song. Large-scale integrated multi-lines optical phased array chip. IEEE Photonics J., 12, 6601208(2020).
[19] F. Xu, X. Ma, Q. Zhang, H. -K. Lo, J. -W. Pan. Secure quantum key distribution with realistic devices. Rev. Mod. Phys., 92, 025002(2020).
[20] G.-W. Zhang, Y.-Y. Ding, W. Chen, F.-X. Wang, P. Ye, G.-Z. Huang, S. Wang, Z.-Q. Yin, J.-M. An, G.-C. Guo, Z.-F. Han. Polarization-insensitive interferometer based on a hybrid integrated planar light-wave circuit. Photonics Res., 9, 2176(2021).
[21] S. M. Sze, K. K. Ng. Physics of Semiconductor Devices(1981).
[22] J.-M. Liu. Photonic Devices(2005).
[23] Z. Lu, Y. Kang, H. Chong, Q. Zhou, H.-D. Liu, J. C. Campbell. Geiger-mode operation of Ge-on-Si avalanche photodiodes. IEEE J. Quantum Electron., 47, 731(2011).
[24] R. E. Warburton, G. Intermite, M. Myronov, P. Allred, D. R. Leadley, K. Gallacher, D. L. J. M. Lever, Z. Ikonic, R. W. Kelsall, E. H. Ceron, A. P. Knights, G. S. Buller. Ge-on-Si single-photon avalanche diode detectors: design, modeling, fabrication, and characterization at wavelengths 1310 and 1550 nm. IEEE Trans. Electron Devices, 60, 3807(2013).
[25] J. D. N. Martinez, G. Michael, C. T. Derose, A. L. Starbuck, P. S. Davids. Single photon detection in a waveguide-coupled Ge-on-Si lateral avalanche photodiode. Opt. Express, 25, 16130(2017).
[26] P. Vines, K. Kuzmenko, J. Kirdoda, D. C. S. Dumas, D. J. Paul, G. S. Buller. High performance planar germanium-on-silicon single-photon avalanche diode detectors. Nat. Commun., 10, 1086(2019).
[27] L. F. Llin, J. Kirdoda, F. Thorburn, L. L. Huddleston, Z. M. Greener, K. Kuzmenko, P. Vines, D. C. S. Dumas, R. W. Millar, G. S. Buller, D. J. Paul. High sensitivity Ge-on-Si single-photon avalanche diode detectors. Opt. Lett., 45, 6406(2020).
[28] F. Thorburn, X. Yi, Z. M. Greener, Z. M. Greener, J. Kirdoda, R. W. Millar, L. L. Huddleston, D. J. Paul, G. S. Buller. Ge-on-Si single-photon avalanche diode detectors for short-wave infrared wavelengths. J. Phys. Photonics, 4, 012001(2022).
[29] S.-Y. Ke, S.-M. Lin, D.-F. Mao, Y.-J. Ye, X.-L. Ji, W. Huang, C. Li, S.-Y. Chen. Design of wafer-bonded structures for near room temperature Geiger-mode operation of germanium on silicon single-photon avalanche photodiode. Appl. Opt., 56, 4646(2017).
[30] Y. Kang, H. X. Lu, Y.-H. Lo. Dark count probability and quantum efficiency of avalanche photodiodes for single-photon detection. Appl. Phys. Lett., 83, 2955(2003).
[31] A. Tomita, K. Nakamura. Balanced, gated-mode photon detector for quantum-bit discrimination at 1550 nm. Opt. Lett., 27, 1827(2002).
[32] C. Park, S. B. Cho, C. Y. Park, S. Baek, S. K. Han. Dual anode single-photon avalanche diode for high-speed and low-noise Geiger-mode operation. Opt. Express, 27, 18201(2019).
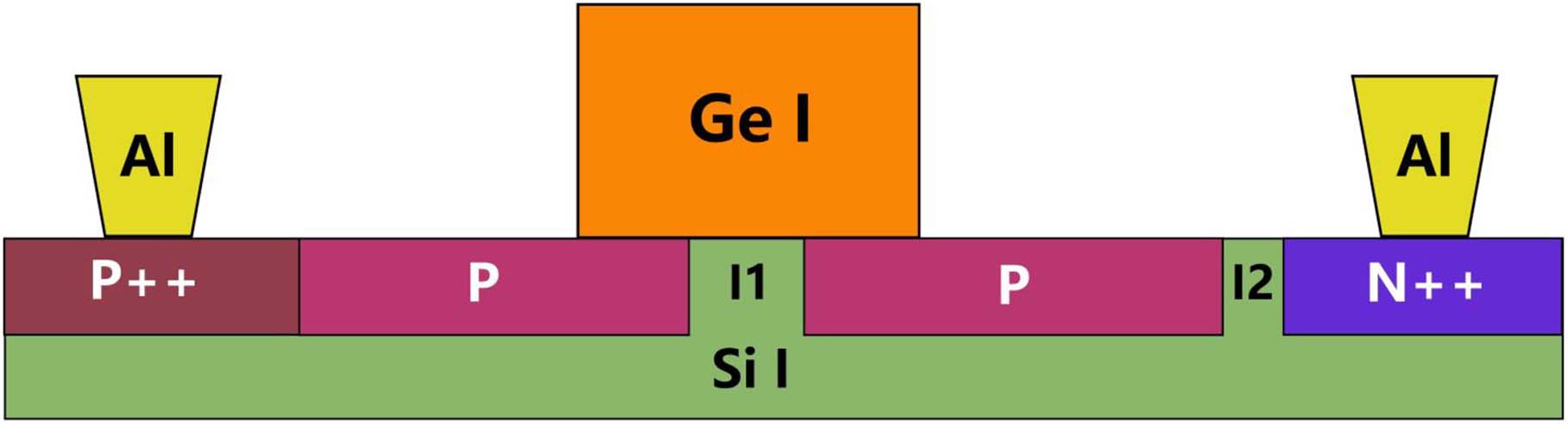
Set citation alerts for the article
Please enter your email address