
- Photonics Research
- Vol. 9, Issue 5, 678 (2021)
Abstract
1. INTRODUCTION
Transparent conductive oxides (TCOs) that function as thin films exhibit high electrical conductivity and good transparency in the visible range [1,2]. Owing to the unique characteristics of TCOs with electrical conductivity, high optical transmittance, and wide bandgap (
In this paper, we prepared ITO films using an electron beam evaporation method and chose two different wavelengths of 1030 nm (out of ENZ region) and 1440 nm (within ENZ region) to analyze the electron transition behavior related to SA and RSA. Our results show conversion behavior from SA to RSA at 1030 nm with increasing incident laser intensity, whereas only SA behavior was observed at 1440 nm. We found that ground-state free electrons bleaching in the conduction band and three-photon absorption play a vital role in this conversion. In the SA process, a large nonlinear absorption coefficient
2. EXPERIMENTS
A. ITO Film Preparation
In this work, ITO films were deposited on fused-silica substrates (
Sign up for Photonics Research TOC. Get the latest issue of Photonics Research delivered right to you!Sign up now
B. Characterization of ITO Films
The surface morphologies of the as-deposited and annealed ITO films were examined by scanning electron microscopy (SEM). The SEM images in Figs. 1(a) and 1(b) show a regular surface with small-sized uniformly distributed particles in both as-deposited and annealed ITO films. The structure properties and the crystalline quality of the films were evaluated by a Bruker AXS/D8 Advance X-ray diffraction (XRD) system (Bruker, Billerica, MA, USA) with Cu
Figure 1.Characterization of as-deposited and annealed ITO films. SEM images showing (a) as-deposited ITO film and (b) ITO film annealed at 400˚C. (c) X-ray diffraction pattern. (d) Linear optical transmittance spectrum.
C. Determination of ENZ Wavelength of ITO Films
To locate the ENZ region of the as-deposited and annealed ITO films, the optical properties of the ITO films were characterized by spectroscopic ellipsometry (HORIBA, UVISEL 2 series, Japan) in the spectral region from 400 to 2000 nm. The dielectric function of the ITO film can be well described using the Drude–Lorentz oscillator model [37]. In ITO film, conduction band electrons have a near continuum of available states, so the conduction band electrons can be treated as a 3D free-electrons gas if we ignore the nonlocal effects. The interaction with an electromagnetic field is described by the Drude model. The Lorentz oscillator model is used to describe the absorption of photons by valence band electrons. According to the Drude–Lorentz oscillator model, the permittivity of ITO film is given by
Figure 2.Real and imaginary components of the permittivity of ITO films. (a) As-deposited ITO film. (b) Post-annealed ITO film.
D. Z-Scan Measurements
Nonlinear optical properties of the ITO films were investigated at 1030 and 1440 nm wavelengths using the femtosecond Z-scan technique developed by Sheik–Bahae [40]. The normalized open aperture (OA) transmittance provides information about the nonlinear absorption, while the normalized closed aperture (CA) transmittance merely represents the nonlinear refraction effect. The schematic of this optical setup is shown in Fig. 3. The films were excited by a fiber laser (1030 nm) and a commercial tunable femtosecond laser (1440 nm) with
Figure 3.Optical nonlinear absorption measurement setup based on the Z-scan method.
3. RESULTS AND DISCUSSION
A. Nonlinear Optical Absorption Properties of As-deposited ITO Films at 1030 nm
In Fig. 4(a), a typical OA Z-scan analysis of an ITO film, measured with 1030 nm laser excitation is shown, which was investigated at input intensities of 15.3, 24.5, 30.6, 45.9, and
Figure 4.Open aperture Z-scan results of as-deposited ITO films under excitation of 1030 nm at different input fluences.
Generally speaking, for ITO film, the nonlinearities can be differentiated into two classes, depending on the relative energies of the bandgap and the optical pump. When an ultrafast optical pulse with energy smaller than the bandgap excites ITO films, the free electrons in the conduction band undergo intraband transitions via free-carrier absorption. However, when the incident fluence is high enough, the interband transitions may occur related to multiphoton absorption. Before excitation, the free electrons in the conduction band are in equilibrium and can be described by a room-temperature Fermi distribution. When the laser pulses excite ITO film, the electrons are energized, and their distribution is highly nonthermal, which produces a redshift of the plasma frequency. We can describe the optical response by means of a phenomenological two-temperature model [43,44]. For optical pulse energies greater than the bandgap or if the excitation intensity is strong enough, the interband transitions occur. The interband transitions increase the total free electron concentration, which causes the blueshift of plasma frequency. In addition, it is worth noting that the nonlocal effects play an important role in the nonlinearity of ultrathin films supporting ENZ and Berreman modes. Hydrodynamic models that account for both nonlinear and nonlocal contributions have been used to explain the nonlinearities and blueshift the plasma frequency of ENZ nanofilms such as ITO and doped-cadmium-oxide films (
The observed SA behavior of ITO films can be attributed to the ground-state free electrons bleaching in the conduction band with anharmonic motion under a strong electromagnetic field excitation (intraband transitions) [22,47]. When the sample moves near the focal point, the enhanced electronmagnetic field inside the sample resonates with the incident light wave, which leads to absorption saturation and the increase of transmittance. With further increase in the laser excitation intensity, the RSA effect was observed, indicating another nonlinear process occurs. It is worth noting that, in the present experiments, the laser photon energy is 1.2 eV (1030 nm) and the bandgap of the ITO film was about
Saturated Absorption Intensity (
Laser Intensity ( | 24.5 | 61.2 | 91.8 | 122.4 | 214.2 |
---|---|---|---|---|---|
64.4 | 67.1 | 76.1 | 105.9 | 88.5 | |
From Table 1, it is interesting to note that
B. Nonlinear Optical Properties of As-deposited ITO Films at 1440 nm
Figures 5(a) and 5(b) show the experimental curves of Z-scan for the as-deposited ITO films under 1440 nm excitation with OA and CA configurations, respectively. In contrast with the results obtained from 1030 nm excitation, only the SA response was observed under 1440 nm excitation, as shown in Fig. 5(a). Obviously, the photon energy corresponding to 1440 nm is
Figure 5.Open aperture Z-scan results of as-deposited ITO films under excitation of 1440 nm at different input fluences. (a) OA Z-scan. (b) CA Z-scan.
To determine the sign and magnitude of the nonlinear refractive index of the as-deposited ITO films, a CA Z-scan measurement was subsequently conducted. The typical shape of the nonlinear refractive index can be extracted from the division of the CA measurement by the OA measurement. The CA/OA curves shown in Fig. 5(b) present a valley peak shape, which means a self-focusing behavior under the illumination of fs laser pulses, indicating a positive value of
C. Tailoring SA and RSA by Thermal Annealing
To further explore the characteristics of electronic nonlinear absorption in the ITO films, we changed the free-carrier concentration in the ITO films via post-annealing treatment. The NLA properties of the ITO films before and after annealing were tested under the same laser excitation conditions (at 1030 and 1440 nm). The intensity-dependent normalized transmittance of the ITO films (as-deposited and annealed) is shown in Figs. 6(a) and 6(b), respectively.
Figure 6.Dependence of normalized transmittance of ITO films (as-deposited and annealed) on optical intensity using different pump sources. (a) 1030 nm. (b) 1440 nm.
Figure 7.Transient response of
Another interesting phenomenon is that the effect of annealing treatment on the resistance of ITO film to laser damage at the two wavelengths is opposite. Obviously, in Fig. 6(a) the damage threshold of the annealed ITO film is reduced when irradiated by the 1030 nm light. In contrast with the results obtained for 1030 nm light excitation, the annealed ITO offers better resistance to laser damage (
4. CONCLUSION
In summary, we systematically investigated the intrinsic mechanisms of NLO absorption in ITO films using the femtosecond Z-scan technique. The results reported in this work indicate that ITO films can exhibit switching between the SA and RSA response, depending on the irradiation wavelength and excitation intensity. Numerical fitting of the experimental data indicates that ground-state free electrons bleaching (third-order nonlinearity) and three-photon absorption (fifth-order nonlinearity) play a significant role in this conversion. The analysis, based on the nonlinear optical absorption model, shows that, under the excitation of a 1440 nm wavelength (within ENZ region), ITO films possess saturable absorption with an imaginary part of the third-order NLO susceptibility of
References
[1] H. Wang, X. Jiao, Q. Liu, X. Xuan, F. Chen, W. Wu. Transparent and conductive oxide films of the perovskite La
[2] J. F. Wager. Transparent electronics. Science, 300, 1245-1246(2003).
[3] C. G. Granqvist, A. Hultaker. Transparent and conducting ITO films: new developments and applications. Thin Solid Films, 411, 1-5(2002).
[4] H. Taha, K. Ibrahim, M. M. Rahman, D. J. Henry, C.-Y. Yin, J.-P. Veder, A. Amri, X. Zhao, Z.-T. Jiang. Sol-gel derived ITO-based bi-layer and tri-layer thin film coatings for organic solar cells applications. Appl. Surf. Sci., 530, 147164(2020).
[5] X. Li, Z. Deng, J. Li, Y. Li, L. Guo, Y. Jiang, Z. Ma, L. Wang, C. Du, Y. Wang, Q. Meng, H. Jia, W. Wang, W. Liu, H. Chen. Hybrid nano-scale Au with ITO structure for a high-performance near-infrared silicon-based photodetector with ultralow dark current. Photon. Res., 8, 1662-1670(2020).
[6] E. W. Li, B. A. Nia, B. K. Zhou, A. X. Wang. Transparent conductive oxide-gated silicon microring with extreme resonance wavelength tunability. Photon. Res., 7, 473-477(2019).
[7] D. C. Adams, S. Inampudi, T. Ribaudo, D. Slocum, S. Vangala, N. A. Kuhta, W. D. Goodhue, V. A. Podolskiy, D. Wasserman. Funneling light through a subwavelength aperture with epsilon-near-zero materials. Phys. Rev. Lett., 107, 133901(2011).
[8] Z. Wang, C. Chen, K. Wu, H. Chong, H. Ye. Transparent conductive oxides and their applications in near infrared plasmonics. Phys. Status Solidi A, 216, 1700794(2019).
[9] M. H. Javani, M. I. Stockman. Real and imaginary properties of epsilon-near-zero materials. Phys. Rev. Lett., 117, 107404(2016).
[10] E. G. Carnemolla, L. Caspani, C. DeVault, M. Clerici, S. Vezzoli, V. Bruno, V. M. Shalaev, D. Faccio, A. Boltasseva, M. Ferrera. Degenerate optical nonlinear enhancement in epsilon-near-zero transparent conducting oxides. Opt. Mater. Express, 8, 3392-3400(2018).
[11] M. Kamandi, C. Guclu, T. S. Luk, G. T. Wang, F. Capolino. Giant field enhancement in longitudinal epsilon-near-zero films. Phys. Rev. B, 95, 161105(2017).
[12] L. Caspani, R. P. Kaipurath, M. Clerici, M. Ferrera, T. Roger, J. Kim, N. Kinsey, M. Pietrzyk, A. Di Falco, V. M. Shalaev, A. Boltasseva, D. Faccio. Enhanced nonlinear refractive index in epsilon-near-zero materials. Phys. Rev. Lett., 116, 233901(2016).
[13] N. Mitoma, S. Aikawa, W. Ou-Yang, X. Gao, T. Kizu, M.-F. Lin, A. Fujiwara, T. Nabatame, K. Tsukagoshi. Dopant selection for control of charge carrier density and mobility in amorphous indium oxide thin-film transistors: comparison between Si- and W-dopants. Appl. Phys. Lett., 106, 042106(2015).
[14] O. Tuna, Y. Selamet, G. Aygun, L. Ozyuzer. High quality ITO thin films grown by dc and RF sputtering without oxygen. J. Phys. D, 43, 055402(2010).
[15] Y. Wang, A. Capretti, L. Dal Negro. Wide tuning of the optical and structural properties of alternative plasmonic materials. Opt. Mater. Express, 5, 2415-2430(2015).
[16] L. Peng, Y. A. Zhao, X. Liu, Z. Cao, D. Li, Y. Lian, Y. Cui, H. Ma, R. Hong, C. Tao, D. Zhang, J. Shao. Tailoring the free carrier and optoelectric properties of indium tin oxide film via quasi-continuous-wave laser annealing. Appl. Surf. Sci., 538, 148104(2021).
[17] Q. Guo, Y. Cui, Y. Yao, Y. Ye, Y. Yang, X. Liu, S. Zhang, X. Liu, J. Qiu, H. Hosono. A solution-processed ultrafast optical switch based on a nanostructured epsilon-near-zero medium. Adv. Mater., 29, 1700754(2017).
[18] J. Guo, H. N. Zhang, C. Zhang, Z. Li, Y. Q. Sheng, C. H. Li, X. H. Bao, B. Y. Man, Y. Jiao, S. Z. Jiang. Indium tin oxide nanocrystals as saturable absorbers for passively Q-switched erbium-doped fiber laser. Opt. Mater. Express, 7, 3494-3502(2017).
[19] Z. M. Zhang, J. J. Liu, Q. D. Hao, J. Liu. Sensitive saturable absorber and optical switch of epsilon-near-zero medium. Appl. Phys. Express, 12, 065504(2019).
[20] Q. H. Xiao, X. Y. Feng, W. Yang, Y. K. Lin, Q. Q. Peng, S. Z. Jiang, J. Liu, L. B. Su. Epsilon-near-zero indium tin oxide nanocolumns array as a saturable absorber for a Nd:BGO laser. Laser Phys., 30, 055802(2020).
[21] P. Guo, R. D. Schaller, J. B. Ketterson, R. P. H. Chang. Ultrafast switching of tunable infrared plasmons in indium tin oxide nanorod arrays with large absolute amplitude. Nat. Photonics, 10, 267-273(2016).
[22] M. Z. Alam, I. De Leon, R. W. Boyd. Large optical nonlinearity of indium tin oxide in its epsilon-near-zero region. Science, 352, 795-797(2016).
[23] N. Kinsey, C. DeVault, J. Kim, M. Ferrera, V. M. Shalaev, A. Boltasseva. Epsilon-near-zero Al-doped ZnO for ultrafast switching at telecom wavelengths. Optica, 2, 616-622(2015).
[24] J. B. Khurgin, M. Clerici, V. Bruno, L. Caspani, C. DeVault, J. Kim, A. Shaltout, A. Boltasseva, V. M. Shalaev, M. Ferrera, D. Faccio, N. Kinsey. Adiabatic frequency shifting in epsilon-near-zero materials: the role of group velocity. Optica, 7, 226-231(2020).
[25] C. Lu, H. Xuan, Y. Zhou, X. Xu, Q. Zhao, J. Bai. Saturable and reverse saturable absorption in molybdenum disulfide dispersion and film by defect engineering. Photon. Res., 8, 1512-1521(2020).
[26] J. Liu, H. Nie, B. Yan, K. Yang, H. Yang, V. Khayrudinov, H. Lipsanen, B. Zhang, J. He. Nonlinear optical absorption properties of InP nanowires and applications as a saturable absorber. Photon. Res., 8, 1035-1041(2020).
[27] X. Feng, J. Liu, W. Yang, X. Yu, S. Jiang, T. Ning, J. Liu. Broadband indium tin oxide nanowire arrays as saturable absorbers for solid-state lasers. Opt. Express, 28, 1554-1560(2020).
[28] R. Wei, H. Zhang, X. Tian, T. Qiao, Z. Hu, Z. Chen, X. He, Y. Yu, J. Qiu. MoS2 nanoflowers as high performance saturable absorbers for an all-fiber passively Q-switched erbium-doped fiber laser. Nanoscale, 8, 7704-7710(2016).
[29] C. Gu, H. Zhang, P. You, Q. Zhang, G. Luo, Q. Shen, Z. Wang, J. Hu. Giant and multistage nonlinear optical response in porphyrin-based surface-supported metal-organic framework nanofilms. Nano Lett., 19, 9095-9101(2019).
[30] Y. Gao, X. Zhang, Y. Li, H. Liu, Y. Wang, Q. Chang, W. Jiao, Y. Song. Saturable absorption and reverse saturable absorption in platinum nanoparticles. Opt. Commun., 251, 429-433(2005).
[31] M. Ali, A. Shehata, M. Ashour, W. Z. Tawfik, R. Schuch, T. Mohamed. Measuring the nonlinear optical properties of indium tin oxide thin film using femtosecond laser pulses. J. Opt. Soc. Am. B, 37, A139-A146(2020).
[32] H. George, J. Reed, M. Ferdinandus, C. DeVault, A. Lagutchev, A. Urbas, T. B. Norris, V. M. Shalaev, A. Boltasseva, N. Kinsey. Nonlinearities and carrier dynamics in refractory plasmonic TiN thin films. Opt. Mater. Express, 9, 3911-3924(2019).
[33] N. Kinsey, A. A. Syed, D. Courtwright, C. DeVault, C. E. Bonner, V. I. Gavrilenko, V. M. Shalaev, D. J. Hagan, E. W. Van Stryland, A. Boltasseva. Effective third-order nonlinearities in metallic refractory titanium nitride thin films. Opt. Mater. Express, 5, 2395-2403(2015).
[34] H. I. Elim, W. Ji, F. Zhu. Carrier concentration dependence of optical Kerr nonlinearity in indium tin oxide films. Appl. Phys. B, 82, 439-442(2006).
[35] J. H. Park, C. Buurma, S. Sivananthan, R. Kodama, W. Gao, T. A. Gessert. The effect of post-annealing on indium tin oxide thin films by magnetron sputtering method. Appl. Surf. Sci., 307, 388-392(2014).
[36] T. A. Gessert, Y. Yoshida, C. C. Fesenmaier, T. J. Coutts. Sputtered In2O3 and ITO thin films containing zirconium. J. Appl. Phys., 105, 083547(2009).
[37] L. Rodriguez-Sune, M. Scalora, A. S. Johnson, C. Cojocaru, N. Akozbek, Z. J. Coppens, D. Perez-Salinas, S. Wall, J. Trull. Study of second and third harmonic generation from an indium tin oxide nanolayer: influence of nonlocal effects and hot electrons. APL Photon., 5, 010801(2020).
[38] Y. L. Hu, X. G. Diao, C. Wang, W. C. Hao, T. M. Wang. Effects of heat treatment on properties of ITO films prepared by rf magnetron sputtering. Vacuum, 75, 183-188(2004).
[39] P. F. Robusto, R. Braunstein. Optical measurements of the surface-plasmon of indium tin oxide. Phys. Status Solidi A, 119, 155-168(1990).
[40] M. Sheikbahae, A. A. Said, T. H. Wei, D. J. Hagan, E. W. Vanstryland. Sensitive measurement of optical nonlinearities using a single beam. IEEE J. Quantum Electron., 26, 760-769(1990).
[41] A. Gnoli, L. Razzari, M. Righini. Z-scan measurements using high repetition rate lasers: how to manage thermal effects. Opt. Express, 13, 7976-7981(2005).
[42] M. Falconieri. Thermo-optical effects in Z-scan measurements using high-repetition-rate lasers. J. Opt. A, 1, 662-667(1999).
[43] M. Clerici, N. Kinsey, C. DeVault, J. Kim, E. G. Carnemolla, L. Caspani, A. Shaltout, D. Faccio, V. Shalaev, A. Boltasseva, M. Ferrera. Controlling hybrid nonlinearities in transparent conducting oxides via two-colour excitation. Nat. Commun., 8, 15829(2017).
[44] M. Conforti, G. D. Valle. Derivation of third-order nonlinear susceptibility of thin metal films as a delayed optical response. Phys. Rev. B, 85, 245423(2012).
[45] M. Scalora, J. Trull, D. de Ceglia, M. A. Vincenti, N. Akozbek, Z. Coppens, L. Rodriguez-Sune, C. Cojocaru. Electrodynamics of conductive oxides: intensity-dependent anisotropy, reconstruction of the effective dielectric constant, and harmonic generation. Phys. Rev. A, 101, 053828(2020).
[46] D. de Ceglia, M. Scalora, M. A. Vincenti, S. Campione, K. Kelley, E. L. Runnerstrom, J. P. Maria, G. A. Keeler, T. S. Luk. Viscoelastic optical nonlocality of low-loss epsilon-near-zero nanofilms. Sci. Rep., 8, 9335(2018).
[47] M. Kauranen, A. V. Zayats. Nonlinear plasmonics. Nat. Photonics, 6, 737-748(2012).
[48] R. del Coso, J. Solis. Relation between nonlinear refractive index and third-order susceptibility in absorbing media. J. Opt. Soc. Am. B, 21, 640-644(2004).
[49] R. W. Boyd, Z. M. Shi, I. De Leon. The third-order nonlinear optical susceptibility of gold. Opt. Commun., 326, 74-79(2014).
[50] D. D. Smith, Y. Yoon, R. W. Boyd, J. K. Campbell, L. A. Baker, R. M. Crooks, M. George. z-scan measurement of the nonlinear absorption of a thin gold film. J. Appl. Phys., 86, 6200-6205(1999).
[51] K. P. Wang, J. Wang, J. T. Fan, M. Lotya, A. O’Neill, D. Fox, Y. Y. Feng, X. Y. Zhang, B. X. Jiang, Q. Z. Zhao, H. Z. Zhang, J. N. Coleman, L. Zhang, W. J. Blau. Ultrafast saturable absorption of two-dimensional MoS2 nanosheets. ACS Nano, 7, 9260-9267(2013).
[52] S. Kumar, M. Anija, N. Kamaraju, K. S. Vasu, K. S. Subrahmanyam, A. K. Sood, C. N. R. Rao. Femtosecond carrier dynamics and saturable absorption in graphene suspensions. Appl. Phys. Lett., 95, 191911(2009).
[53] A. Mayer, F. Keilmann. Far-infrared nonlinear optics. II.
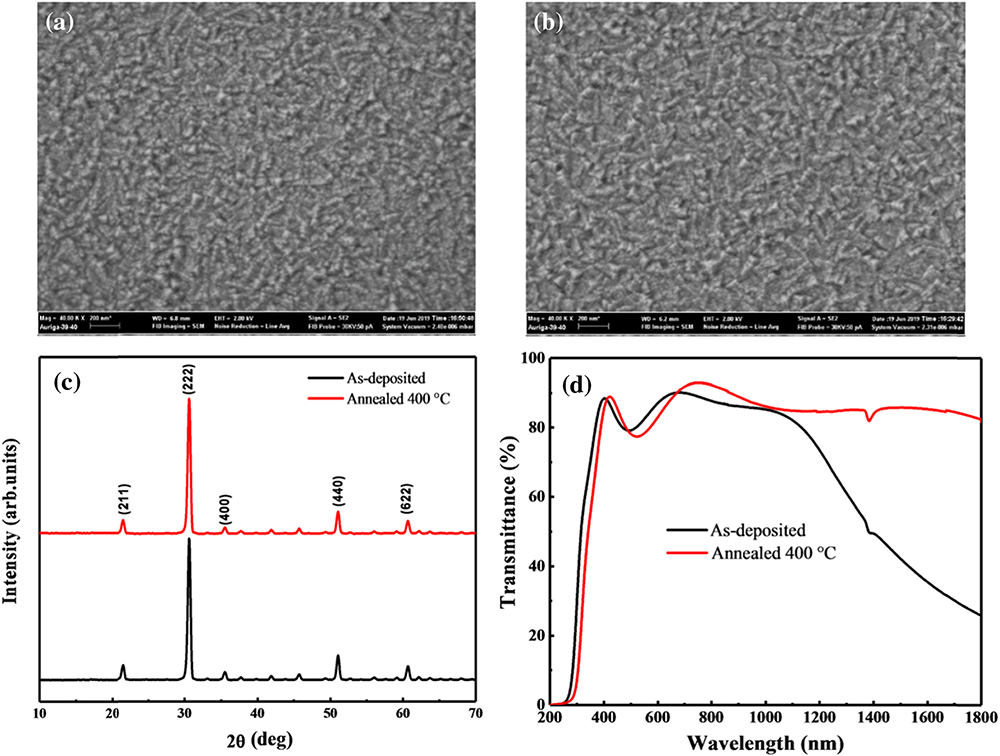
Set citation alerts for the article
Please enter your email address