
- Journal of Inorganic Materials
- Vol. 35, Issue 7, 839 (2020)
Abstract
In recent decades, organic dyes have been widely used in many industrial production process, such as clothing, food processing, medicine, spray and rubber manufacturing industries[
Graphitic carbon nitride (g-C3N4), a non-metal polymeric visible-light driven photocatalyst with suitable band gap (around 2.7 eV) and valence band (around 1.5 eV) has received intense interest in photocatalysis community owing to its excellent chemical stability, relatively low cost and non-toxicity[
Bismuth vanadate (BiVO4) possesses relatively narrow bandgap (~2.43 eV) and valence band (~2.75 eV), high chemical stability and remarkable energy conversion, and can act as a photocatalyst to degrade organic pollutants[
In this work, a new photocatalyst with a ternary heterojunction, BiVO4/GO/g-C3N4, was prepared by a two-step method. The binary composite GO/g-C3N4 was synthesized by impregnation-calcination method and combined with BiVO4 to form a ternary heterojunction. The photocatalytic performance of the ternary system (BiVO4/ GO/g-C3N4), the binary system (GO/g-C3N4) and single systems (g-C3N4 and BiVO4) under visible-light radiation, was systematically evaluated by Rhdamine B degradation, and the mechanism of photocatalytic reaction of the Z-scheme heterojunction BiVO4/GO/g-C3N4 was discussed in detail.
1 Experimental
1.1 Materials and synthesis
Dicyandiamide, bismuth nitrate pentahydrate (Bi(NO3)3·5H2O), ammonium metavanadate (NH4VO3), were purchased from Aladdin Chemical Reagent Co. Ltd (Shanghai, China). Graphene oxide (GO) powder was purchased from Suzhou Hengqiu Graphene Co., Ltd., China.
1.1.1 Preparation of the GO/g-C3N4
GO/g-C3N4 binary photocatalytic heterojunction was prepared by an impregnation-calcination method according to previous report with some modifications[
1.1.2 Preparation of the BiVO4/GO/g-C3N4 ternary photocatalyst
Firstly, 0.7 mmol Bi(NO3)3·5H2O and 0.7 mmol NH4VO4 were completely dissolved in 10 mL 2 mol/L HNO3 aqueous solution and 10 mL deionized water, respectively. Secondly, different amounts of GO/g-C3N4 were added to Bi(NO3)3 mixture solution and dispersed by 0.5 h stirring and ultrasound, then NH4VO4 aqueous solution was put into the above mixture solution. In order to get the precursor, the resulting pale yellow suspension was vigorous stirred for 1 h at room temperature. Then it was poured into a 50 mL Teflon-lined autoclave, heated at 180 ℃ for 7 h, and naturally cooled to room temperature. The obtained precipitate was washed with deionized water and absolute ethanol for 3 times, respectively. Finally, the samples were dried at 60 ℃ for 4 h. The composite photocatalysts having different mass ratios of BiVO4 to GO/g-C3N4 were recorded as 8 : 2, 6 : 4, 4 : 6 and 2 : 8 (the former is BiVO4 occupancy). Here the photocatalytic heterojunctions were denoted as B8GC2, B6GC4, B4GC6, B2GC8, respectively according to these different mass ratios. Meanwhile, for comparison, pure BiVO4 was also prepared in the same manner without the addition of GO/g-C3N4.
1.2 Characterizations
X-ray diffraction (XRD) patterns were characterized on a D/Max-2500 X-ray diffractometer (Rigaku, Japan) with Cu-Kα radiation at a scan rate (2θ) of 0.05 (°)/s in the range of 3°-70°. The microstructures were examined by a transmission electron microscope (TEM) (Hitachi H7650, HITACHI, Japan) and high-resolution transmission electron micrographs (HRTEM) (TecnaiG2 F20, FEI, America). The sizes and morphologies were collected with a field emission scanning electron microscope (Gemini SEM500, ZEISS, Germany). UV-visible absorbance spectra of the dry-pressed disk samples were obtained by means of a UV-visible spectrophotometer (UV-2600, Shimadzu, Japan) in the range of 200-800 nm with BaSO4 as a reflectance standard. The chemical bonding status of the samples were analyzed on an FT-IR spectro-meter (FTIR-650, Gangdong Technology, China). The specific surface area of the samples were measured through a mesoporous surface physical adsorber (NOVA4200e, Quantachrome, America). Photoluminescence (PL) spectra were measured under 315 nm excitation wavelength by a Fluorescence Spectrophotometer (F380, Gangdong Technology, China).
1.3 Photoelectrochemical measurements
All photoelectrochemical studies were carried out by an electrochemical workstation (CHI760E, Chenhua, China) in a standard three-electrode system which used the as-prepared samples as the working electrodes, the platinum wire as the counter electrode, and Ag/AgCl electrode as the reference electrode. 0.1 mol/L Na2SO4 aqueous solution was used as the electrolyte. The working electrodes were prepared as follows: 0.2 g photocatalyst was ground and 0.5 mL anhydrous ethanol with the ultrasonic dispersion to make uniform suspension slurry. Then, the obtained slurry was smeared onto a 2 cm×2 cm F-doped SnO2- coated conducting glass (FTO glass) using spincoating strategy for twice. Photocurrent curves were measured using an Amperometric I-t curves method under a 30 s intermittent irradiation, and the initial voltage was 1.2 V. Electrochemical impedance spectra (EIS) were obtained in the frequency range of 0.01-100,000 Hz at initial voltage of 0 V.
1.4 Evaluation of photocatalytic activity
A 500 W Xe lamp (Beijing AuLight, China) was utilized as a visible-light source with 400 nm cut-off filters. The degradation of RhB was used as the evaluation standard for the photocatalytic ability of the sample. The photocatalytic degradation products of RhB were analyzed using an UV-Vis spectrophotometer (UV-2600, SHIMADZU, Japan) at 553 nm.
In the photocatalytic performance evaluation experiment, 10 mg catalysts was added into the RhB (20 mL, 20 mg·L-1) each time. In order to reach adsorption-desorption equilibrium, the solution was stirred for 30 min in the dark before the irradiation. Suspensions with dosage of 4.0 mL were withdrawn and analyzed regularly. After suspensions were centrifuged (8000 r/min, 10 min) for photocatalyst removal, detected at 553 nm absorbance by UV-Vis spectrophotometer.
The photocatalytic degradation efficiency (E) was obtained for measurement and calculation according to Eq. (1):
Where C is the concentration of RhB at different time, C0 is the initial concentration at the RhB adsorption equilibrium, A and A0 are the corresponding absorption values.
2 Results and discussion
2.1 Optical, structural and morphological characteristics
The reflective patterns of as-prepared composite samples were studied by powder XRD to infer the phase structures. As shown in Fig. 1(a), the strongest diffraction (002) peak near 2θ=27.4° corresponds to an interplanar distance of the conjugated aromatic system of 0.326 nm, which reflects the interlayer tight accumulation of aromatic rings. Another apparent additional (001) peak relates to an in-plane structural packing motif which interlayer space is 0.672 nm[
Figure 1.XRD patterns of BiVO4, g-C3N4/GO, B2GC8, and BiVO4/ GO/g-C3N4 (a), and FT-IR spectra of the as-prepared GO, GO/ g-C3N4, BiVO4, BiVO4/GO/g-C3N4 (b)
In addition, compared to the crystalline structures of pure BiVO4, no matter whether it is the shape or position, the added compound has little effect on the characteristic peak of pure BiVO4. Therefore, BiVO4 in heterojunction maintains a stable crystal structure, which isn’t affected by the introduction of GO/g-C3N4. Due to no introduction of other new peaks, the combination of BiVO4 and GO/g-C3N4 don’t generate impurities, revealing that GO, g-C3N4 and BiVO4 coexist in the ternary nanocomposite photocatalysts.
FT-IR spectra (Fig. 1(b)) show that graphite oxide exhibits strong hydroxyl stretching vibration absorption peak near 3477 cm-1. The C=O stretching vibration peak on the carboxyl group of graphene oxide appears at 1790 cm-1. The absorption peak at 1685 cm-1 may be the absorption peak due to the bending vibration of C-OH[
No other peaks were observed in BiVO4/GO/g-C3N4 spectrum, probably due to the reduction of graphene oxide during the calcination. Vibration band at 739 cm-1 is generated by ѵ(VO43-) stretching vibration[
To examine chemical composition and states of samples, the prepared photocatalysts were analyzed by XPS characterization (Fig. 2). It is obvious that the detection spectrum of the BiVO4/GO/g-C3N4 can determine that the sample contains only Bi, V and O elements for BiVO4, and C, N and O elements for GO/g-C3N4, and no impurities (Fig. 2(a)). According to the Gaussian curve-fitted signal deconvolution, the high-resolution N1s spectrum of BiVO4/GO/g-C3N4 has N species in different chemical environments (Fig. 2(b)). The highest N1s peak at 398.6 eV corresponds to sp2-hybridized nitrogen (C=N-C). Two other weak N1s peaks locating at 399.8 and 401.5 eV can be assigned to tertiary nitrogen (N-(C)3) and amino functional groups (C-N-H), respectively. The weak C1s peak (284.6 eV) in the BiVO4 sample spectrum was produced by the XPS instrument itself contaminating hydrocarbons, whereas the peak of the GO/g-C3N4 sample at 288.08 eV was attributed to the sp2-hybridized carbon in N-containing aromatic ring (N-C=N), which positively shifted to 288.38 eV for the BiVO4/GO/g-C3N4 sample in Fig. 2(c)[
Figure 2.XPS survey scans of BiVO4, GO/g-C3N4 and BiVO4/ GO/g-C3N4(a), and high-resolution spectra of N1s (b), C1s (c), Bi1s (d) in BiVO4, GO/g-C3N4 and BiVO4/GO/g-C3N4, respectively
TEM images (Fig. 3(a, b) show that the layered g-C3N4 tightly anchors on the wrinkled surface of the GO to form a stable heterojunction in the GO/g-C3N4 composite. After hydrothermal reaction, 1-5 µm BiVO4 crystal particles uniformly distributed on the surface of GO/g-C3N4, and a ternary photocatalyst was synthesized. In Fig. 3(c), the pure BiVO4 crystal in the shape of decagon and polyhedron is monoclinic, which is consistent with the results of XRD. It can be observed that the pure BiVO4 particles own average size of 1-5 µm, while due to the introduction of GO/g-C3N4 in the system, BiVO4 crystals become more delicate and reduce to the size of 100- 500 nm (Fig. 3(d)), which may be affected by the chemical environment on the surface or edge of GO/g-C3N4, such as hydroxyl and carbonyl that could easily limit the nucleation and growth process of the BiVO4 crystal. The layered structure of GO/g-C3N4 may include stacking layers, which provides a site for the production and growth of BiVO4 particles, and are in accordance with the XRD results.
Figure 3.TEM images of the as-prepared GO/g-C3N4 (a) and BiVO4/GO/g-C3N4(b), and SEM images of BiVO4 (c) and BiVO4/GO/g-C3N4 (d)
The optical property of semiconductor photocatalysts plays a significant role in investigating the energy band structure for the photocatalytic activity. The UV-Vis diffuse reflectance spectra (DRS) of the obtained samples were revealed in Fig. 4(a). The absorption edge of the as-synthesized pure g-C3N4 is at about 556 nm, while a broader absorption edge is at about 550 nm, suggesting that BiVO4 can effectively utilize visible light for photocatalysis. The addition of GO to the GO/g-C3N4 composite increases the background absorption of the sample, allowing the sample to more fully utilize visible-light as compared with pure g-C3N4. Moreover, B2GC8 exhibits similar absorption characteristics to pure BiVO4, indicating that the binding of GO/g-C3N4 only on the surface of BiVO4 did not change the original lattice structure, which is consistent with XRD and SEM results. The widest visible light absorption of the ternary BiVO4/GO/ g-C3N4 composites show that BiVO4, g-C3N4 and GO generate effective synergy.
Figure 4.UV-Vis adsorption spectra of prepared samples (a) and calculated band gap of BiVO4 and g-C3N4 (b)
The band gaps were elevated by the following Eq. (2):
Where α, h, ν, and A are absorption coefficient, Planck constant, light frequency, and a constant, respectively. The n value is determined by the type of optical transition of semiconductors (n=1 for direct transition and n=4 for indirect transition). BiVO4 belongs to the direct transition semiconductors but g-C3N4 direct transition semiconductors. Through data processing and analysis, the Eg values of BiVO4 (2.43 eV) and g-C3N4 (2.7 eV) can be obtained respectively (Fig. 4(b)).
2.2 Photocatalytic activity analyses
The visible light photocatalytic degradation spectra of the RhB with obtained photocatalysts is showed in Fig. 5(a). It is apparent that g-C3N4 and BiVO4 exhibit poor photocatalytic degradation of RhB solution activity under visible light owing to faster photogenerated electron/hole pair recombination and relatively narrow absorption of visible light regions, which is the same as the UV-Vis diffuse reflectance spectra.
Figure 5.Photocatalytic degradation of RhB (a) and dynamics of RhB photodegradation reaction (ln(
Although g-C3N4 and BiVO4 respond to visible light, the effect is not significant, and the degradation efficiencies after irradiation for 120 min under visible light are 17% and 22%. As contrasted with g-C3N4 and BiVO4, the photocatalytic degradation efficiency of RhB by GO/g-C3N4 and B2GC8 composites is significantly improved, reaching 51% and 85%, respectively. The specific surface areas of g-C3N4, BiVO4, GO/g-C3N4 and B2CG8 are 7.6, 1.7, 5.7, 3.2 m2·g-1, respectively. The calculated reaction rates per unit area were 0.197, 1.17, 0.68, 3.29 mg·h-1·m-2, respectively. The degradation efficiency of the ternary BiVO4/GO/g-C3N4 composite is higher than those of other catalysts, and the photoluminescence (PL) intensity of the ternary heterojunction was very low. The above results indicate that the Z scheme ternary photocatalyst has an effective redox capability and an excellent charge separation driving force to degrade RhB. The comparison results show that the Z-scheme ternary heterojunction has significant photocatalytic activity under visible light in terms of degradation of RhB.
Fig. 5(b) shows that the photocatalytic decomposition of RhB is supposed to follow a pseudo-first-order kinetics reaction, and the evaluating formula is expressed as Eq. (3):
Where Kapp is the apparent rate constant (min-1), C0 and Ct is the RhB concentration at reaction time 0 and t, respectively.
2.3 Photoelectrochemical analyses
In order to obtain more evidence that Z-scheme heterojunction promotes photocatalytic degradation of dyes, samples electrodes are recorded for several on-off cycles of irradiation. As can be seen from Fig. 6(a), the I-t curve of the synthetic sample was analyzed by a 30 s interval dark and visible light illumination cycle. When visible light is irradiated, the photocurrent value is gradually stabilized, and when the light is turned off, the photocurrent rapidly drops to zero, and this process can be cyclically reproduced. The performance indicates that the generated electrons move in the direction of the visible light, thereby generating a certain intensity of photocurrent. In the present case, the photocurrent diagram of B2GC8 ternary heterojunction and GO/g-C3N4 binary composites reveals an improved photocurrent response than that of the single BiVO4, which indicates excellent charge separation efficiency. This apparent enhancement of photocurrent also demonstrates that due to spatial isolation of photogenerated carriers at the interface between BiVO4, GO and g-C3N4, photogenerated electron-hole pairs achieves lower recombination and more efficient separation for the GO/g-C3N4 composites. The reduced species in the electrolyte are trapped or captured the hole on the BiVO4 surface, while the electrons are effectively transported to g-C3N4 by GO sheets.
Figure 6.Transient photocurrent responses (a), photoluminescence (PL) spectra (b), and EIS Nyquist plots (dot) (c) of g-C3N4, BiVO4, GO/g-C3N4 and BiVO4/GO/g-C3N4
Photoluminescence (PL) analysis was carried out to reveal the intensity of the fluorescence emitted by the recombination of photogenerated electrons and the holes, thus inferring the process of migration, separation and recombination of photogenerated electrons and holes in catalyst. It is obvious that at an excitation wavelength of 315 nm, BiVO4 is excited to a very strong steady-state fluorescence intensity, and the GO/g-C3N4 composite is weaker than that of BiVO4, which is attributed to the fact that GO can act as an electronic repository to facilitate charge transfer and inhibit charge recombination in Fig. 6(b). The B2GC8 composite was excited to have the weakest fluorescence intensity, indicating that it has a lowest recombination rate of photogenerated carriers under visible-light irradiation. This can be mainly attributed to the transfer of electrons excited on BiVO4 to the valence band of g-C3N4 through GO, while the holes on g-C3N4 are transferred to the conduction band of BiVO4 through GO, thereby achieving spatial isolation of the two carriers. As a result to some extent, direct recombination of electrons and holes was prevented.
Electrochemical impedance (EIS) is an effective method for analyzing the improved charge separation efficiency, which is also conducted in Fig. 6(c). It depicted the magnitude of the impedance arc diameter under sample illumination, g-C3N4>BiVO4>GO/g-C3N4> B2GC8, indicating that the B2GC8 ternary coupled photocatalyst has the ability to rapidly transfer and separate photogenerated carriers. This result is consistent with the effect of the RhB degradation experiment.
2.4 Photocatalytic mechanism analyses
Through the above characterization results and analysis, the potential photocatalytic mechanism of the Z-scheme BiVO4/GO/g-C3N4 ternary photocatalyst was tentatively proposed, and the reaction process was illustrated in Fig. 7. Due to the energy band gap of 2.43 and 2.7 eV, BiVO4 and g-C3N4 can be excited even under visible light irradiation, resulting in electron hole separation. According to the different conduction band and valence band position of the BiVO4 and g-C3N4, g-C3N4 and BiVO4 anchored on GO by strong interfacial electrostatic interaction to form a Z-scheme heterojunction. Under the interface Z-scheme heterostructure, the photogenerated electrons in the CB of BiVO4 spontaneously move to the VB g-C3N4 and BiVO4. The interface Z-scheme heterostructure results in spatial segregation of photogenerated carriers and then accumulates electrons and holes. The electrons in the CB of g-C3N4 respectively generate ·OH radicals, and ·O2- has strong oxidation, and then induce RhB degradation together with the holes. Therefore, the photocatalytic performance of the g-C3N4/GO/BiVO4 ternary heterostructure can be significantly enhanced.
Figure 7.Possible photocatalytic enhanced mechanism over BiVO4/GO/g-C3N4 Z-scheme photocatalysts under visible light irradiation
3 Conclusions
A new Z-scheme photocatalyst of ternary BiVO4/GO/g-C3N4 composites was successfully synthesized and employed for the photocatalytic degradation of RhB. In the photocatalytic process of BiVO4/GO/g-C3N4, GO nanosheet act as fast transmission channels between BiVO4 and g-C3N4 and can suppress electron-hole recombination, which significantly promotes the charge separation and improves the redox ability of the ternary heterojunction. As a result, about 85% of RhB dye was degraded by the ternary composites after 120 min visible light irradiation, superior to that of the single or binary system. In addition, the enhanced degradation efficiency could be ascribed to the active h+ species in degradation progress in trapping experiment. Overall, the Z-scheme BiVO4/GO/g-C3N4 is an excellent photocatalyst with high-efficiency and has good potential for further application to organic-pollutants decomposition.
References
[6] T MASSEY A, R GUSAIN, S KUMARI et al. Hierarchical microspheres of MoS2 nanosheets: efficient and regenerative adsorbent for removal of water-soluble dyes. Industrial & Engineering Chemistry Research, 55, 7124-7131(2016).
[17] Q WU, S BAO, B TIAN et al. Double-diffusion-based synthesis of BiVO4 mesoporous single crystals with enhanced photocatalytic activity for oxygen evolution. Chem. Commun.(Camb), 52, 7478-7481(2016).
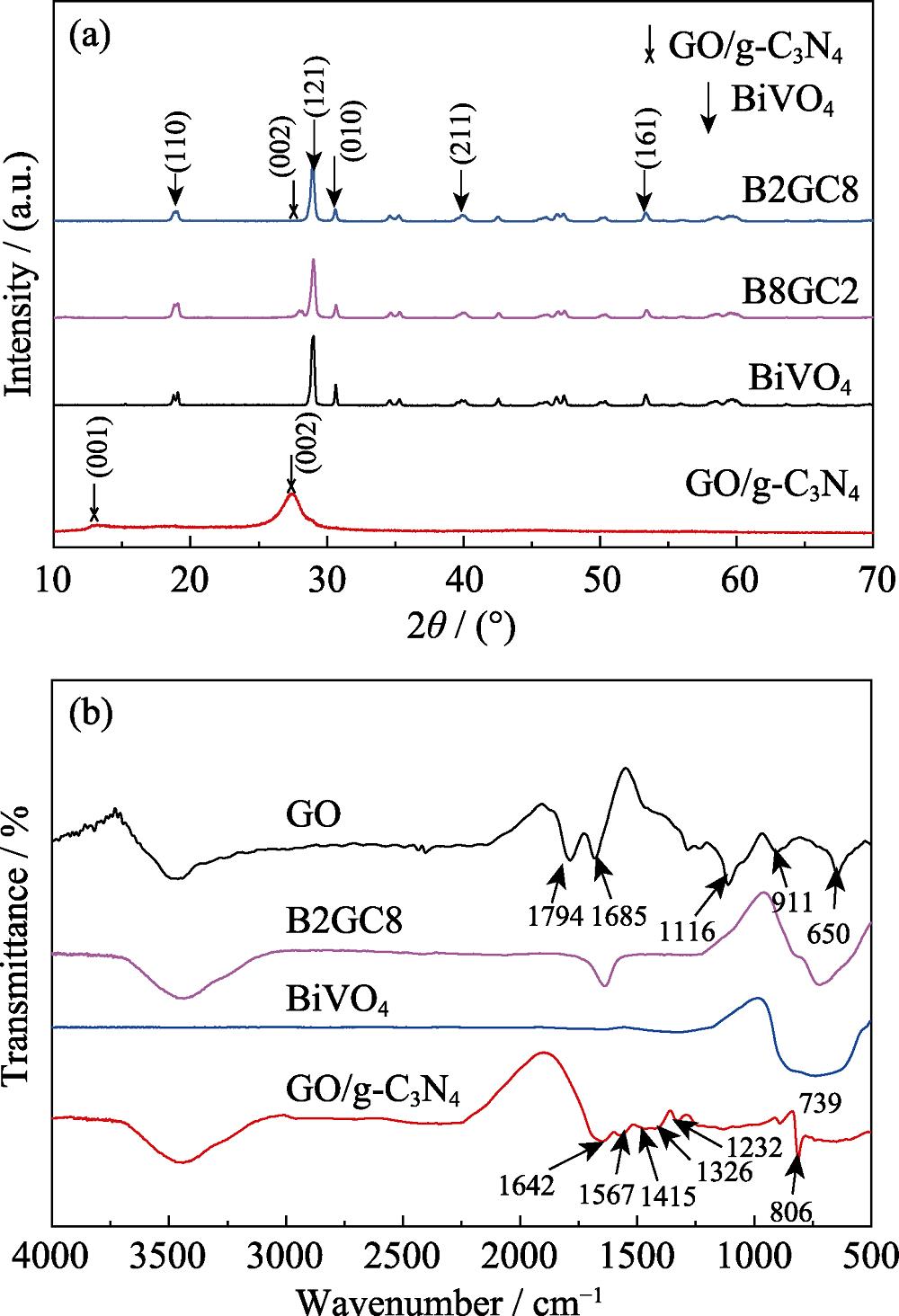
Set citation alerts for the article
Please enter your email address