Optical fiber surface plasmon resonance (SPR) sensors point toward promising application potential in the fields of biomarker detection, food allergen screening, and environmental monitoring due to their unique advantages. This review outlines approaches in improving the fiber SPR sensing performance, e.g., sensitivity, detection accuracy, reliability, cross-sensitivity, selectivity, convenience and efficiency, and corresponding sensing applications. The sensing principles of SPR sensors, especially the performance indicators and their influencing factors, have been introduced. Current technologies for improving the fiber SPR performance and their application scenarios are then reviewed from the aspects of fiber substrate, intrinsic layer (metal layer), and surface nanomaterial modification. Reasonable design of the substrate can strengthen the evanescent electromagnetic field and realize the multi-parameter sensing, and can introduce the in situ sensing self-compensation, which allows corrections for errors induced by temperature fluctuation, non-specific binding, and external disturbances. The change of the intrinsic layer can adjust the column number, the penetration depth, and the propagation distance of surface plasmon polaritons. This can thereby promote the capability of sensors to detect the large-size analytes and can reduce the full width at half-maximum of SPR curves. The modification of various-dimensionality nanomaterials on the sensor surfaces can heighten the overlap integral of the electromagnetic field intensity in the analyte region and can strengthen interactions between plasmons and excitons as well as interactions between analyte molecules and metal surfaces. Moreover, future directions of fiber SPR sensors are prospected based on the important and challenging problems in the development of fiber SPR sensors.

- Photonics Research
- Vol. 10, Issue 1, 126 (2022)
Abstract
1. INTRODUCTION
Abbreviation | Full name |
2-D | Two-dimensional |
3-D | Three-dimensional |
AET | 2-aminoethanethiol |
AgNW-OF | Ag nanowires and tapered optical fiber |
ATR | Attenuated total reflection |
AuNPs | Au nanoparticles |
BP | Black phosphorus |
BSA | Bovine serum albumin |
CNTs | Carbon nanotubes |
CPWR | Coupled plasmon waveguide resonance |
Cs/PSS | Chitosan/polysodium styrene sulfonate |
CuNPs | Cuprum nanoparticles |
DA | Detection accuracy |
DE | Distal ends |
DML | Dielectric matching layer |
ELP | Electroless plating |
ERY | Erythromycin |
EW | Evanescent wave |
FOM | Figure of merit |
FWHM | Full width at half-maximum |
GNP | Graphene nanoplatelet |
GO | Graphene oxide |
HCF | Hollow-core fiber |
HGNPs | Hollow gold nanoparticles |
IgG | Immunoglobulin G |
ITO | Indium tin oxide |
LOD | Limit of detection |
LOQ | Limit of quantification |
LPFG | Long period fiber grating |
LRSPPs | Long range surface plasmon polaritons |
LRSPR | Long range surface plasmon resonance |
LS | Lateral surface |
LSPR | Localized surface plasmon resonance |
MMF | Multi-mode fiber |
MWCNTs | Multi-walled carbon nanotubes |
NGWSPR | Nearly guided wave surface plasmon resonance |
PBA | Pyrene-1-boronic acid |
PCF | Photonic crystal fiber |
PDA | Polydopamine |
PDMS | Polydimethylsiloxane |
PdNPs | Palladium nanoparticles |
PMBA | P-mercaptophenylboronic acid |
PtNPs | Platinum nanoparticles |
PTOF | Polymer-tipped optical fiber |
QF | Quality factor |
rGO | Reduced graphene oxide |
RI | Refractive index |
SEM | Scanning electron microscopy |
SERS | Surface enhanced Raman scattering |
SLR | Surface lattice resonance |
SMF | Single-mode fiber |
SNR | Signal to noise ratio |
SPF | Side-polished fiber |
SPPs | Surface plasmon polaritons |
SPR | Surface plasmon resonance |
SWCNTs | Single-walled carbon nanotubes |
TE | Transverse electric |
TFBG | Tilted fiber Bragg grating |
TM | Transverse magnetic |
TMDCs | Transition metal dichalcogenides |
WCSPR | Waveguide coupled surface plasmon resonance |
XO | Xanthine oxidase |
Table 7. Nomenclature
Surface plasmon resonance (SPR) sensing methods can realize analyses of biomolecular interactions with high sensitivity, rapid response, and no labeling [1–3]. Optical fiber-based SPR effect can be regarded as the nonlinear multiple integral of the prism-based SPR effect at the incident angle and the incident plane. Optical fiber SPR sensors possess the advantages of anti-electromagnetic interference, corrosion resistance, small volume, and great flexibility, and they can easily form a sensor network through cascading or other manners. This allow us to achieve the real-time, online, remote, and multi-parameter detection of target analytes [4,5]. Therefore, the research on fiber SPR sensors has made significant progress in the field of biochemical sensing. However, on the one hand, the size of viruses, cells, proteins, nucleic acids, and some other biological macromolecules is in the order of micrometers or even millimeters and is much larger than the penetration depth of surface plasmon polaritons (SPPs), which is on a scale of hundreds of nanometers [6,7]. This indicates that the above substances cannot be detected sensitively via conventional SPR sensors. On the other hand, the full width at half-maximum (FWHM) of conventional SPR sensors is relatively large, which exceeds 100 nm, and will result in a low detection accuracy (DA). Furthermore, the detection repeatability and the anti-interference capability of conventional SPR sensors need to be improved. Therefore, performance improvement approaches for fiber SPR sensors have been widely studied and are mainly categorized into the following three branches.
Reasonable and flexible design of fiber substrates by means of cascading, polishing, and other methods can increase the evanescent field leakage and can introduce the in situ interference self-compensation function. This will promote the capability of sensors in detecting the variety and the intensity of external factors.
Sign up for Photonics Research TOC. Get the latest issue of Photonics Research delivered right to you!Sign up now
Adjusting intrinsic layers (metal layers) of fiber SPR sensors can modulate the original light field, which makes sensors suitable for different application scenarios. Attenuated total reflection (ATR)-based long range SPR (LRSPR) [8,9], coupled plasmon waveguide resonance (CPWR) [10,11], nearly guided wave SPR (NGWSPR) [11], and waveguide coupled SPR (WCSPR) [12] can be considered as optimization parameters to improve the performance of conventional SPR sensors from different aspects.
The modification of various-dimensionality nanomaterials with high refractive index (RI) on the sensor surfaces by physical sputtering or chemical bond coupling can cause large disturbance of the evanescent field [9,13], can strengthen the confined electric field intensity [11], and can promote the capability of sensors for detecting small changes in the ambient environment.
According to above discussions, an overview of performance improvement approaches for fiber SPR sensors and their sensing applications is presented. There have been some reviews on SPR, but they are different from our report in terms of research scope and focus. In 2011, Shalabney
Optical fiber SPR sensors based on commercial fibers, customized fiber structures, and metastructure platform have been outlined in Refs. [14–19], but they are more focused on the classification of fiber SPR sensors. Meanwhile, applications of fiber SPR sensors in various scenarios (e.g., environmental monitoring, food safety detection, and biological immunoassay) have also been involved in Refs. [15,16,20–23]. Both classification and application scenarios are obviously different from the performance improvement approaches discussed in our paper. In addition, the sensing mechanism and applications of specific types of fiber SPR sensors (e.g., D-type fiber SPR sensors, hybrid plasmonic fiber sensors, molecular imprinting improved fiber SPR sensors, and surface plasmon cavities) have been systematically reviewed in Refs. [24–30]. The focuses of these reviews are particular, which are different from the broader focuses of our paper. We hope that this review can provide an insightful overview of fiber SPR sensors and inspirations for the development of SPR sensors.
2. THEORETICAL FOUNDATION OF FIBER SPR SENSORS
A. Sensing Mechanism of Fiber-Based SPR
The disassembly of cylindrical surfaces in the optical fiber allows the SPR phenomenon at each point to be explained by a three-layer structure. As shown in Fig. 1(a), the layer configuration of a traditional SPR sensor consists of a substrate layer, an intrinsic layer, and a dielectric layer. The substrate can employ commercial fibers (e.g., multi-mode fiber (MMF) [32], photonic crystal fiber (PCF) [33], hollow-core fiber (HCF) [34]), customized fiber such as suspended-core fiber [35], and the fiber grating (e.g., tilted fiber Bragg grating (TFBG) [5], long period fiber grating (LPFG) [36]). The intrinsic layer utilizes materials, with negative real-part and positive imaginary-part in dielectric constants, such as mono/bimetallic film with high reflectivities and low absorption rates (e.g., Au [37], Ag [38], Cu [39], Al [40]). The dielectric layer refers to the analyte layer.
Figure 1.(a) The layer configuration and (b) resonance spectrum of SPR sensor. (c) Field/current distributions of the insulator-metal-insulator model corresponding to SPR sensing structure [31]. Note: I, II, and III are three modes with the lowest loss; red line, black line, and orange arrow represent electric field distribution, magnetic field distribution, and current conduction, respectively.
The total reflection will occur when the transverse magnetic (TM) polarized light is projected on the substrate-metal layer interface at an angle larger than the critical value. The resulted evanescent wave (EW) enters the metal layer, and its energy decreases exponentially. This excites SPPs to propagate along the upper surface of the metal layer [7,11]. When wave vector components of the EW and SPPs on the interface are equal, SPPs will be strongly coupled with the EW. At this point, most of the energy of the incident light is coupled into SPPs [14] while a resonance dip appears in the transmission spectrum, as shown in Fig. 1(b). This phenomenon is called SPR. The SPP mode, which is strongly coupled to the EW, is called the resonance SPP mode, i.e., SPR mode. The SPP mode that is weakly coupled to the evanescent wave is called the non-resonance SPP mode. The wavelength of the incident light, which corresponds to the occurrence of the strong coupling, is defined as the resonance wavelength (
B. Performance Indicators of Fiber SPR Sensors and Their Influencing Factors
The performance improvement of fiber SPR sensors mainly is targeted at some indicators, and this section introduces the typical performance indicators of the sensor and their influencing factors. Since it is not convenient to adjust the incident angle at the fiber core-cladding interface, most fiber SPR sensors are designed based on the wavelength scanning. The depth of the resonance dip is determined by the energy coupling between the resonance SPP modes and the evanescent wave. The stronger the coupling is, the deeper the resonance dip will be. The FWHM of resonance dip is determined by the difference between the resonance SPP modes-evanescent wave coupling and the non-resonance SPP modes-evanescent wave coupling. The greater the difference is, the narrower the FWHM will be. Meanwhile, some researchers claim that the observed SPR curve band is the result of the convolution of the resonance dips excited by separate optical modes [41], and the Ohmic loss of metal and the radiative loss of SPPs on the parallel axis [in Fig. 1(c)] lead to the broadening of the resonance dip [11].
The sensitivity (
The limit of detection (LOD) of the sensor represents the minimum concentration or the quantity of the target components that can be detected from the test sample within a given degree of reliability. Equations (6)–(8) [46–48] display the different ways to evaluate LOD. Slightly different from LOD, the limit of quantification (LOQ) represents the lowest detectable analyte concentration near blank considering the standard deviation of calibration curve, as expressed in Eq. (9) [46]. In general, the kinetic adsorption curve, acquired from biomass detection by using the sensor, is obtained by fitting the Langmuir adsorption isotherm equation [49] or logistic regression equation [50]. The sensitivity (
Parameter Indices to Evaluate the Performance of SPR Sensors
Index | Equation | Parametric Meaning | Ref. |
---|---|---|---|
[ | |||
DA | – | ||
SNR | [ | ||
FOM | – | [ | |
QF | – | ||
[ | |||
LOD | |||
LOQ | [ |
3. PERFORMANCE IMPROVEMENT APPROACHES FOR FIBER SPR SENSORS AND THEIR SENSING APPLICATIONS
A. Reasonable Design of Fiber Substrate to Improve Sensor Performance
1. Increasing the Evanescent Field Leakage to Amplify SPR Signal
The use of different commercial optical fibers as the substrate to construct SPR sensors will obtain different sensing characteristics. PCF-based SPR sensors possess high sensitivity due to the high confinement loss of SPPs excited by a Gaussian-like leaky mode of the PCF element [15]. HCF-based SPR sensors can realize all-fiber distributed sensing, since the entire inner wall of the fiber can be coated with chemicals to form the sensing area [34]. Fiber grating-based SPR sensors can obtain a smaller FWHM and a higher SNR [36].
SPR is the electromagnetic resonance stimulated by the evanescent field leakage when the total reflection occurs at the substrate interface. Therefore, research works are carried out to increase the evanescent field leakage for enhancing the sensitivity by customizing the fiber structure through micro/nano processing (e.g., fused tapering [52], side polishing [53], bending into U-shape [54]). In 2014, Cennamo
Figure 2.(a) Schematic of the sensing structure and (b) concentration response as well as (c) time response spectra for the nicotine detection using the tapered plastic fiber SPR sensor [55]. (d) Schematic of the sensing structure and (e) glucose concentration response as well as (f) temperature response spectra of the SPF-SPR sensor [56]. (g) Schematic of the sensing structure and (h) human IgG concentration response spectra of the U-shaped fiber SPR sensor [57].
When the cladding and even the core of the fiber on one side are polished away, the evanescent field energy will leak from the polished area. This allows the side-polished fiber (SPF)-based SPR sensor to have a higher sensitivity [53]. In 2009, Tsai
2. Multi-Parameter Sensing
The signal spectral response of the fiber SPR sensor has a wide wavelength range; and thus, multi-parameter sensing can be realized by capturing different central wavelengths in different bands [59,60]. The implementations are mainly categorized into the following three branches. First, multiple detection lanes are constructed based on different fiber substrates [61–65]. In 2021, Xiao
Figure 3.(a) Schematic of the PCF-SPR sensor for the simultaneous measurement of magnetic field, RI, and temperature [61]. (b) Loss spectrum of the PCF-SPR sensor for the measurement of magnetic field [61]. (c) Schematic of the experimental setup for the characterization process of the SPR fiber tip sensor [66]. (d) Transmission spectrum of the SPR fiber tip sensor for the measurement of the liquid level [66]. (e) Schematic of the LRSPR sensor and experimental setup for the simultaneous measurement of RI and temperature [67]. (f) Transmission spectrum of the LRSPR sensor for the measurement of temperature [67].
Second, double detection lanes are constructed in the same sensing area [66,70]. In 2019, Alonso-Murias
Thirdly, multi-parameter measurement is carried out based on the sensitive characteristics of dielectric materials [67,71]. In 2020, Wang
The optical fiber sensors used for multi-parameter measurement can meet the needs of different complex scenarios, which is of great significance to application.
3. Self-Compensation for Temperature Fluctuation
Multi-parameter measurement is generally used to solve the cross-influence among parameters. In 2019, Zhang
The relationship between the wavelength shift in the optical grating resonance peak (
According to Eq. (12), the resonance wavelength shift caused by the change in the concentration and the temperature of the glucose can be calculated separately. Meanwhile, the SPR sensing unit and the LPFG temperature compensation unit were set close to each other, and thus the sensor could realize the in situ temperature self-compensation in the detection of the glucose concentration.
Similarly, Weng
4. Self-Compensation for Non-Specific Binding
When the fiber SPR sensor is employed to detect the biomass based on the antigen-antibody specific binding, the resonance wavelength shift caused by the interaction between the antigen and the non-specific binding site on the sensor surface will affect the detection reliability. In 2021, Wang
Similarly, Wang
B. Attenuated Total Reflection-Based SPR Modes
1. Long Range SPR
LRSPR refers to an electromagnetic resonance mode stimulated by adding a lossless dielectric buffer layer (e.g., Cytop [9], Teflon [77],
Figure 4.(a) Layer configuration of LRSPR sensor. (b) Schematic of the sensing structure and (c) resonance spectrum for the detection of different BSA concentrations in the
2. Coupled Plasmon Waveguide Resonance and Nearly Guided Wave SPR
The CPWR sensor incorporates a thick (typically 500 nm [11]) dielectric layer with high-complex permittivity (e.g., ITO [83], ZnO [80],
Similar to CPWR, NGWSPR can be stimulated by coating a thin (typically 10 nm [11]) dielectric layer with high real part of the dielectric constant (e.g.,
3. Waveguide Coupled SPR
WCSPR refers to an electromagnetic resonance mode generated by coating an additional metal layer on the surface of the waveguide layer, based on CPWR. The layer configuration is shown in Fig. 4(g). When the light ray is incident at a small angle, the SPPs propagating along the interface between the lower metal layer and the waveguide layer will be excited by the TM polarized light. With the increase of the incident angle, the SPPs on the interface between the upper metal layer and the waveguide layer will also be excited [43]. Due to the coupling between the SPR and the waveguide resonance modes generated by the interference between multiple rays, narrow resonance dips and interference valleys that do not shift with the change of the external RI appear in the transmission spectrum [81]. In 2019, Ma
4. Comparison of ATR-Based SPR Modes
Due to unique sensing characteristics of ATR-based SPR modes, corresponding sensors are suitable for different applications, e.g., stimulating stronger surface enhanced Raman scattering (SERS) signal for pH sensing [87] and obtaining biological information regarding the cellular micromotion [88] and the DNA hybridization [89]. Different types of ATR-based SPR modes are summarized in Table 2 for a comparison.
Comparison of Different ATR-Based SPR Modes
ATR-Based | Layer Configuration | Polarization State of Excitation Light | Advantage | Disadvantage |
---|---|---|---|---|
SPR | Substrate/metal layer/analyte | TM | The layer configuration is simple, and the sensitivity is high. | The FWHM is wide, and the DA is low. |
LRSPR | Substrate/dielectric layer with permittivity is pure real-number and lower than that of substrate/metal layer/analyte | TM | The FWHM is narrow, the DA is high, and the sensor is suitable for biomacromolecules detection. | The sensitivity depends heavily on symmetric configuration. |
CPWR | Substrate/metal layer/thick waveguide layer with high-complex permittivity/analyte | TM or TE | The FWHM is narrow, and the DA is high. | The sensitivity is low. |
NGWSPR | Substrate/metal layer/thin waveguide layer/analyte | TM | The sensitivity is high, and the resonance dip is deep. | The DA is low. |
WCSPR | Substrate/metal layer/waveguide layer/metal layer/analyte | TM or TE | The sensitivity is slightly higher, and the sensor possesses self-reference function. | The layer configuration is complex. |
C. Applications of Various-Dimensionality Nanomaterials in Fiber SPR Sensing
1. Zero-Dimensional Nanomaterials
Zero-dimensional nanomaterials are confined in the nanoscale range (1–100 nm) in all three directions, and their electrons cannot move freely, e.g., nanospheres [90] and nanoparticles [91]. In 2020, Wang
Figure 5.(a) Fabrication process in the SPP coupling-based fiber biosensor [75]. (b) Variation of resonance wavelength for human IgG detection [75]. (c) Schematic of the fiber SPR sensor fabricated by PDA accelerated ELP for immunoassay. Inset, scanning electron microscopy (SEM) image of the Au seeds formed Au layer [92]. (d) Sensitivity fitting curve of the sensor for detecting different concentrations of human IgG [92]. (e) Fabrication process in the HGNPs modified fiber LRSPR biosensor [51]. (f) Resonance spectrum for human IgG detection [51].
Due to the assistance of the coupling between the inner and the outer walls, the hollow gold nanoparticles (HGNPs) have a stronger plasmonic field compared to solid GNPs [93]. In 2021, Cheng
Based on the above discussion, typical application examples of zero-dimensional nanomaterials applied in improving the SPR sensing performance are listed in Table 3.
Application Examples of Zero-Dimensional Nanomaterials in SPR Sensing
Sensing Structure | Target Analyte | Simulated/Experimental Results | Ref. | ||
---|---|---|---|---|---|
Sensitivity | LOD | Notes | |||
Fiber/Au layer/AuNPs/analyte | RI 1.3332–1.3710 | 3074.34 nm/RIU | – | – | [ |
Fiber/Au seeds formed Au layer/PDA/anti-IgG/IgG | RI 1.333–1.359/1.359–1.386 | 2054/3980 nm/RIU | – | The FOM of the sensor was | [ |
Human IgG 2–100 μg/mL | 0.41 nm/(μg/mL) | 0.90 μg/mL | |||
Fiber/PDA/Au seeds formed Au layer/PDA/anti-IgG/IgG | RI 1.328–1.386 | 1391–5346 nm/RIU | – | – | [ |
Human IgG 0.5–40 μg/mL | 0.65 nm/(μg/mL) | 0.22 μg/mL | |||
Fiber/DML/Au layer/PDA-HGNPs/anti-IgG/IgG | Human IgG 1–40 μg/mL | 1.84 nm/(μg/mL) | 0.20 μg/mL | DML refers to dielectric matching layer. Combination of LRSPR and electric field coupling effects. The spike-and-recovery for serum samples detection was 107.62%. | [ |
Fiber/Au layer-PMBA/glucose/AuNPs-AET-PMBA | Glucose | – | 80 nM | PMBA and AET refer to p-mercaptophenylboronic acid and 2-aminoethanethiol, respectively. | [ |
PCF/Au layer/GO/anti-IgG/AuNPs-IgG | RI 1.3323–1.3359 | 13,592.36 nm/RIU | Synergistic sensitization of zero-dimensional AuNPs and two-dimensional graphene oxide (GO). | [ | |
Human IgG 1–35 μg/mL | 1.36 nm/(μg/mL) | 0.015 μg/mL | |||
Fiber core/Au | Goat-anti-IgG 5–25 μg/mL | 0.054 μg/mL | Synergistic sensitization of zero-dimensional AuNPs and two-dimensional | [ | |
Fiber core/Ag layer/ERY imprinted nanoparticles | ERY | 0.205 nm/nM | 1.62 nM | ERY refers to erythromycin. The spike-and-recovery for real samples detection was 98.2%–102.0%. | [ |
Fiber/Ag core- | 2 nm/lg[M] | The LOD for serum samples was | [ | ||
Fiber/triangular AgNPs/GO/analyte | RI 1.3318–1.3495 | 1114.80 nm/RIU | – | The apices generated greater electric field amplification. | [ |
Fiber/Au nanostars arrays | SERS | – | – | The apices generated greater electric field amplification, and the proposed sensor was demonstrated with 45 times electric field intensity enhancement compared with Au nanorods design. | [ |
2. One-Dimensional Nanomaterials
One-dimensional nanomaterials are confined in the nanoscale range in two directions and are extended to the macroscale range in the last direction. Their electrons can only move freely in one direction, e.g., nanotubes [38], nanorods [104], nanowires [105], and nanofibers [106]. Carbon nanotubes (CNTs) have been widely applied in the fields of biochemical sensing, nano-optics, and photothermal conversion owing to their extraordinary chemical, electrical, and optical characteristics. In 2021, Pathak
Figure 6.(a) Schematic of the PdNPs embedded/PPy shell coated MWCNT-based fiber SPR probe and the laboratorial setup. Inset, schematic and SEM image of the PdNP embedded/PPy shell coated MWCNTs and SEM image of the fiber probe surface [38]. (b) Resonance spectrum obtained by detecting hydrazine with different concentrations [38]. (c) The fabrication process of the double-layer Au nanorods and GO sensitized PCF-SPR sensor [104]. (d) Resonance spectrum obtained by detecting human IgG with different concentrations [104]. (e) Schematic of the
The modification of Au nanorods on the surface of SPR sensor can effectively enhance the sensitivity of the sensor because of the transverse and the longitudinal plasmon electric field coupling between nanorods and the Au layer [91]. In 2020, Xia
Based on the above discussion, typical application examples of one-dimensional nanomaterials applied in improving the SPR sensing performance are listed in Table 4.
Application Examples of One-Dimensional Nanomaterials in SPR Sensing
Sensing Structure | Target Analyte | Simulated/Experimental Results | Ref. | |||
---|---|---|---|---|---|---|
Sensitivity | FOM | LOD | Notes | |||
Fiber core/ITO/Si/SWCNTs/analyte | RI 1.330–1.335 | 9780 nm/RIU | – | ITO and SWCNTs refer to indium tin oxide and singlewalled CNTs, respectively. | [ | |
Fiber core/Au layer/MWCNTs-PtNPs/analyte | RI 1.3385–1.3585 | 5923.14 nm/RIU | – | Synergistic sensitization of zero-dimensional platinum nanoparticles (PtNPs) and one-dimensional MWCNTs. | [ | |
Fiber/Au layer/MWCNTs-CuNPs/analyte | Nitrate | 3.25 nm/lg[M] | – | – | Simultaneous measurement of two parameters. Synergistic sensitization of zero-dimensional cuprum nanoparticles (CuNPs) and one-dimensional MWCNTs. | [ |
Temperature | – | – | ||||
Fiber core/Ag layer/MWCNTs-CuNPs/analyte | Nitrate | 0.08062 nm/nM | – | 4 nM | Synergistic sensitization of zero-dimensional CuNPs and one-dimensional MWCNTs. | [ |
Fiber core/Ag layer/PdNPs-PPy-MWCNTs/analyte | Hydrazine | 0.09 nm/nM | – | 20 nM | Synergistic sensitization of zero-dimensional PdNPs and one-dimensional MWCNTs. The spike-and-recovery rate of real sample detection was 97.5%–102.8%. | [ |
Fiber core/Ag layer/MWCNTs/analyte | Sulfamethaxazole | – | 891.80 nM | – | [ | |
Fiber core/Ag layer/PPy-MWCNTs/analyte | Dopamine | 68.58 nm/lg[M] | – | 0.0189 nM | – | [ |
Fiber core/Ag layer/graphene-MWCNTs-poly-(methyl methacrylate)/analyte | Methane gas 10–100 ppm (parts per million) | – | – | – | Synergistic sensitization of one-dimensional MWCNTs and two-dimensional graphene. The maximum shift in the resonance wavelength was 30 nm for methane gas detection. | [ |
Fiber core/Ag | Xanthine | 0.0262 nm/nM | – | 12.70 nM | The sensor worked well for the detection of xanthine in green tea samples. | [ |
Fiber core/Ag layer/ZnO: graphene nanofibers/analyte | Nicotine | – | 74 nM | The sensor worked well for the detection of nicotine in cigarette samples. | [ | |
PCF/Au layer/Au nanorods/GO/Au nanorods/analyte | RI 1.3323–1.3361 | 22,248.22 nm/RIU | – | Synergistic sensitization of one-dimensional Au nanorods and two-dimensional GO. | [ | |
Human IgG 1–15 μg/mL | 3.28 nm/(μg/mL) | – | 6.10 ng/mL | |||
PCF/Au layer/double-layer Au nanorods/GO/analyte | RI 1.3320–1.3366 | 25,642.65 nm/RIU | – | Synergistic sensitization of one-dimensional Au nanorods and two-dimensional GO. | [ | |
Human IgG 1–15 μg/mL | 4.35 nm/(μg/mL) | – | 4.60 ng/mL | |||
U-bent fiber/Au layer/ITO nanorods/graphene/analyte | RI 1.3330–1.3634 | 690.70 nm/RIU | – | – | Synergistic sensitization of one-dimensional ITO nanorods and two-dimensional graphene. | [ |
DNA 0.1–100 nM | – | – | 0.10 nM | |||
PCF/Ag nanowires/analyte | RI 1.33–1.38 | 9314.28 nm/RIU | – | [ | ||
PCF/Au nanowires/analyte | RI 1.32–1.38 | 10,286 nm/RIU | – | [ | ||
Fiber/bimetallic nanowire gratings/analyte | RI 1.33–1.49 | 643.75 nm/RIU | – | – | – | [ |
3. Two-Dimensional Nanomaterials
Two-dimensional nanomaterials are confined in the nanoscale range in one direction and are expanded to the macroscale range in other two directions. Their electrons can only move freely in such two directions. Two-dimensional nanomaterials popularly used for improving the performance of SPR sensors are listed in Table 5. Graphene possesses lower energy losses (e.g., Ohmic loss and radiative loss), high electron transport mobility, and high surface-to-volume ratio, and it can accommodate more organic and biological molecules because of the
Popular Two-Dimensional Materials for SPR Sensor Performance Improvement
Category | Basic Chemical Formula | Ensample |
---|---|---|
Graphene and derivatives | – | GO, reduced GO (rGO) |
Phosphorene | – | Black phosphorus (BP), BlueP |
Transition metal dichalcogenides (TMDCs) | ||
MXene | ||
Perovskite | ||
Notes | M, X, Y, Z, and |
Different two-dimensional layered materials can be vertically stacked together and integrated to form van der Waals heterostructures and superlattices. This can manipulate optical and electronic characteristics of their interlayer interactions, to provide technical support for the development of next-generation devices [126]. In 2019, Rahman
Figure 7.(a) Schematic of the phosphorene-graphene/TMDC heterostructure-based fiber SPR biosensor [127]. (b) Resonance spectrum of the biosensor for DNA hybridization detection [127]. (c) Schematic of the
MXene can realize the attractive biosensing due to its chemical stability, fully functionalized surface termination, high metal conductivity, tunable direct bandgap, small work function, large adsorption energy, and hydrophilic biocompatible surface [123,126,131]. In 2020, Chen
Based on the above discussion, typical application examples of two-dimensional nanomaterials applied in improving the SPR sensing performance are listed in Table 6.
Application Examples of Two-Dimensional Nanomaterials in SPR Sensing
Sensing Structure | Target Analyte | Simulated/Experimental Results | |||||
---|---|---|---|---|---|---|---|
Sensitivity | FOM | LOD | Notes | Ref. | |||
Fiber core/Ag layer/Pt layer/ITO/graphene/analyte | RI 1.33–1.36 | 4150 nm/RIU | – | – | [ | ||
Fiber core/Ag layer/GO/analyte | Glucose adulterant | 21,140 nm/RIU | – | – | [ | ||
Fructose adulterant | 18,890 nm/RIU | – | |||||
Fiber core/Ag layer/Au | RI 1.3318–1.3701 | 3061.84 nm/RIU | – | – | [ | ||
Fiber core/Au | BSA 10–50 μg/mL | 0.9234 nm/(μg/mL) | – | 0.29 μg/mL | – | [ | |
RI 1.3314–1.3623 | 6184.40 nm/RIU | – | – | [ | |||
Human IgG 5–70 μg/mL | 1.014 nm/(μg/mL) | – | |||||
Fiber core/Cr layer/Au | RI 1.333–1.358 | 2793.36 nm/RIU | – | – | [ | ||
Goat-anti-rabbit IgG | – | – | 0.33 μg/mL | ||||
Fiber core/Al layer/ | RI 1.330–1.332 | 6200 nm/RIU | – | – | – | [ | |
SPF/Cr layer/Au | Glucose | 6708.87 nm/RIU | – | – | PBA refers to pyrene-1-boronic acid. | [ | |
Graphene | 4050 nm/RIU | – | 2-D materials refer to two-dimensional materials. The sensor was also utilized successfully to detect DNA hybridization. | [ | |||
Fiber core/Au layer/BP/2-D materials/analyte | RI 1.33–1.39 | 3950 nm/RIU | – | ||||
3975 nm/RIU | – | ||||||
3975 nm/RIU | – | ||||||
4000 nm/RIU | – | ||||||
Fiber core/NaF layer/Ag layer/BlueP/2-D materials/analyte | – | – | – | [ | |||
– | – | ||||||
Fiber core/Ag layer/SnSe/analyte | RI 1.33–1.37 | 3475 nm/RIU | – | – | – | [ | |
Fiber core/Ag layer/GNP- | Dopamine 0–100 μM | 10.66 nm/μM | – | 0.031 μM | GNP refers to graphene nanoplatelet. | [ | |
Fiber core/Ag | Acetylcholine 0–10 μM | 8.709 nm/μM | – | 0.038 μM | – | [ | |
Heterocore fiber/Au | RI 1.3343–1.3658 | 2180.20 nm/RIU | – | – | – | [ | |
Fiber core/Au | RI 1.333–1.335 | 3725 nm/RIU | – | – | [ | ||
PTOF/Au | RI 1.333–1.343 | 6710 nm/RIU | – | – | – | [ | |
SPF/Au | RI 1.3332–1.3710 | 2543.33 nm/RIU | – | – | – | [ | |
RI 1.3710–1.4140 | 6040.42 nm/RIU | – | – |
4. Three-Dimensional Nanomaterials
Three-dimensional nanomaterials represent composites composed of one or more basic structural units from zero-dimensional, one-dimensional, and two-dimensional materials, e.g., three-dimensional superlattice structures [146,147] and nanostructured metal architectures [148,149].
In 2020, Li
Figure 8.(a) Preparation procedure of the three-dimensional composite-based fiber LSPR biosensor [150]. (b) Schematic of the three-dimensional composite on the fiber surface, transmission electron microscopy image of Au nanoparticles covered by multilayer graphene, and schematic of the DNA detection process [150]. (c) Real-time wavelength redshift for DNA detection [150]. (d) Schematic of the bioreceptor patterning onto the Au coated fiber surface using DNA nanotechnology: three-dimensional DNA lateral surface (LS) origami, distal ends (DE) origami, and tetrahedron. (Dark green, bioreceptors; dark gray spheres, thiol groups; light green and red, ssDNA [151].) (e) Calibration curves for thrombin bioassay on the fiber SPR biosensing platform [151].
Based on the quantum size effect, the surface effect, and the dielectric confinement effect, nanomaterials with new functions are expected if there are further breakthroughs in the structure, the composition, the arrangement, and the size of three-dimensional nanomaterials.
4. FUTURE DIRECTIONS OF DEVELOPMENT
A. End-Face Reflective Sensing Structure
Compared with the transmission-type optical fiber SPR sensor [152] shown in Fig. 9(a), the end-face reflective optical fiber SPR sensor can be inserted into a small piece of sample or cramped space to directly acquire the signal in the dip-and-read way. Combined with mature fiber communication technology, this can achieve the high-efficiency, low-damage, and invasive measurement. At present, there are mainly three types of end-face reflective fiber SPR sensing probes [14], as shown in Fig. 9(a). On the cylindrical surface at the end of fiber, the cladding is removed, and a metal layer is coated to stimulate the SPR. Meanwhile, the end-face of fiber is also coated with the metal to serve as a mirror. Moreover, when the light is reflected from the end-face and enters the fiber again, the optical path is doubled, which enhances the plasmon resonance effect. In 2019, Kong
Figure 9.(a) Transmission-type fiber SPR sensor [152] based on core mismatch I and reflective fiber SPR sensor [14] based on flat tip II, tapered tip III, and angle polished tip IV. (b) Schematic of the sensing structure of the protruding-shaped fiber plasmonic microtip probe and the testbed [153]. (c) SEM image of the microtip probe and schematic of the bio-probe [153]. (d) Langmuir adsorption curve and (e) sensitivity fitting line for human IgG detection with different concentrations [153].
B. Combination of Nanoarray Structure and Fiber SPR Sensing
A highly ordered plasmonic nanoarrays, produced by self-assembly technology [154], focused ion/electron beam lithography [155], and two-photon laser direct writing [37], can stimulate various coupling optical effects such as Fano resonance [156], guided mode resonance [157], surface lattice resonance (SLR) [158], and SERS [154]. The combination of above effects and the SPR sensing can further strengthen the light-matter interaction, can reduce the radiative loss, and can thus improve the sensor performance. The periodic nanoarray also provides tunable structure parameters, wider spectral range, and higher degree of freedom. The SPR sensor based on the nanoarray has higher sensitivity and FOM, which expands the application scenarios of SPR. Furthermore, it is compatible with existing imaging equipment, microfluidic chips, and photonic circuits so that the fabricated devices can be highly integrated and miniaturized. Based on the above advantages [157], nanoarray structures have attracted more and more attention.
In 2016, He
Figure 10.(a) Optical micrograph of the plasmonic crystal cavity on the SMF end-face [159]. (b) Resonance spectrum of the SPR device for the detection of different solutions [159]. (c) Process in the fabrication of nanotriangular arrays on the reflective fiber SPR sensor end-face based on colloidal lithography technology and the SEM image of the nanotriangular arrays [160]. (d) Sensitivity fitting lines for the RI detection of the Au triangularly patterned and non-patterned sensors [160]. (e) Block diagram of the nanotrimer arrays on the bent fiber end-face and the SEM image of the nanotrimer arrays [161]. Resonance spectra of the (f) SLR-based and (g) LSPR-based sensors [158].
In 2018, Wang
C. Plasmonic Antenna Integrated on Tapered Fiber Tip
Lab on tip based probe devices provide high-precision dynamic tuning of plasmonic antenna and high-resolution detection through the strong interaction between plasmonic hotspots and samples. The test objects include the molecular adsorption or deformation [162], molecular vibration spectrum [163], quantum tunneling [164,165], etc.
As shown in Figs. 11(a) and 11(b), the coupling between the tapered fiber (or dielectric waveguide) and metal nanowires (or SPP waveguide) can greatly improve the light wave coupling and sub-wavelength focusing efficiency of the near-field optical microscope probe. This realized the scanning Raman spectroscopy imaging with a spatial resolution of 1 nm [166,169]. As shown in Fig. 11(c), it was observed by Danckwerts
Figure 11.(a) Near-field optical microscope probe based on the high-efficiency coupling of Ag nanowires and tapered optical fiber (AgNW-OF) [166]. (b) Polarization-resolved k-space imaging of the light emitted from the nanofocused SPP mode at the AgNW-OF probe tip [166]. (c) The four-wave-mixing produced signal increased sharply when the Au nanoparticle-fiber probe approached another Au nanoparticle [167]. (d) The molecular fluorescence changed from enhancement to quenching when the Au nanoparticle-fiber probe approached a single molecule [168].
D. Initial Conceptions
Although the fiber SPR sensors can realize the detection of physical and biochemical parameters, there is still some space to improve fiber SPR sensors in terms of accuracy, cross-sensitivities, selectivity, costs, and reliability. In the future research, it will be promising to involve microelectromechanical systems [170,171] for improving the stability of the device and to employ liquid metals [172] for developing flexible optical devices. The integrated and miniaturized design of fiber SPR sensors can utilize the two-photon three-dimensional micro/nano film printing to achieve the accurate, real-time, and on-site detection [171,173]. Terahertz biosensors based on metamaterials can also be vigorously developed to achieve the non-invasive detection for biomarkers or biochemical indicators in living organisms [156,173]. According to the sensing characteristics of fiber SPR sensors modified by different nanomaterials, nanomaterials can be reversely designed at the atomic level to modify sensors to obtain desired sensing characteristics, e.g., to locate the resonance valley in the optical band. In order to improve the performance of fiber SPR sensors from different aspects, various approaches can be jointly applied in the implementation of sensors. For example, the modification of nanomaterials on the surface of the LRSPR sensor with improved metal layer quality can enhance the sensitivity of the sensor while ensuring the DA [174,175].
5. CONCLUSION
This review work summarized current major approaches for improving the performance of fiber SPR sensing and the applications of the manufactured sensors mainly in the field of biochemical detection. The sensing principle and the performance indicators including determinants of SPR sensors were demonstrated to clarify the research direction of the performance improvement. The application examples and the internal mechanisms in the SPR sensing performance improvement were reviewed from three aspects, corresponding to the basic layer configuration of fiber SPR sensors, namely substrate, intrinsic layer, and surface nanomaterial modification. Relevant contents were summarized and compared in detail. In general, the performance improvement of fiber SPR sensors mainly lies in heightening the overlap integral of the SPP electric field intensity on the sensor surface to enhance the sensitivity, reducing the energy losses of SPP to narrow down the FWHM of SPR curve and to improve the DA and the SNR, and introducing the sensing self-compensation to enhance the detection reliability. Furthermore, the development direction of fiber SPR sensors was discussed based on the state of the art in plasmonic detection technologies.
References
[1] A. Jebelli, F. Oroojalian, F. Fathi, A. Mokhtarzadeh, M. de la Guardia. Recent advances in surface plasmon resonance biosensors for microRNAs detection. Biosens. Bioelectron., 169, 112599(2020).
[2] A. Koponen, E. Kerkela, T. Rojalin, E. Lazaro-Ibanez, T. Suutari, H. O. Saari, P. Siljander, M. Yliperttula, S. Laitinen, T. Viitala. Label-free characterization and real-time monitoring of cell uptake of extracellular vesicles. Biosens. Bioelectron., 168, 112510(2020).
[3] G. Y. Wang, Y. Lu, L. C. Duan, J. Q. Yao. A refractive index sensor based on PCF with ultra-wide detection range. IEEE J. Sel. Top. Quantum Electron., 27, 5600108(2021).
[4] H. X. Yu, Y. Chong, P. H. Zhang, J. M. Ma, D. C. Li. A D-shaped fiber SPR sensor with a composite nanostructure of MoS2-graphene for glucose detection. Talanta, 219, 121324(2020).
[5] W. J. Hu, Y. Y. Huang, C. Y. Chen, Y. K. Liu, T. A. Guo, B. O. Guan. Highly sensitive detection of dopamine using a graphene functionalized plasmonic fiber-optic sensor with aptamer conformational amplification. Sens. Actuators B, 264, 440-447(2018).
[6] P. Berini. Long-range surface plasmon polaritons. Adv. Opt. Photon., 1, 484-588(2009).
[7] J. Homola. Surface plasmon resonance sensors for detection of chemical and biological species. Chem. Rev., 108, 462-493(2008).
[8] J. Y. Jing, Q. Wang, W. M. Zhao, B. T. Wang. Long-range surface plasmon resonance and its sensing applications: a review. Opt. Laser Eng., 112, 103-118(2019).
[9] C. T. Yang, L. Wu, P. Bai, B. Thierry. Investigation of plasmonic signal enhancement based on long range surface plasmon resonance with gold nanoparticle tags. J. Mater. Chem. C, 4, 9897-9904(2016).
[10] J. Y. Ma, K. Liu, J. F. Jiang, T. H. Xu, S. Wang, P. X. Chang, Z. Zhang, J. H. Zhang, T. G. Liu. All optic-fiber coupled plasmon waveguide resonance sensor using ZrS2 based dielectric layer. Opt. Express, 28, 11280-11289(2020).
[11] A. Shalabney, I. Abdulhalim. Sensitivity-enhancement methods for surface plasmon sensors. Laser Photon. Rev., 5, 571-606(2011).
[12] H. T. Zhang, Y. J. Geng, S. P. Xu, W. Q. Xu, Y. Tian, J. Yu, W. Y. Deng, B. Yu, Y. Liu. Surface plasmon field-enhanced Raman scattering based on evanescent field excitation of waveguide-coupled surface plasmon resonance configuration. J. Phys. Chem. C, 124, 1640-1645(2020).
[13] J. Y. Jing, Q. Zhu, Z. X. Dai, S. Y. Li, Q. Wang, W. M. Zhao. Sensing self-referenced fiber optic long range surface plasmon resonance sensor based on electronic coupling between surface plasmon polaritons. Appl. Opt., 58, 6329-6334(2019).
[14] Y. Zhao, R. J. Tong, F. Xia, Y. Peng. Current status of optical fiber biosensor based on surface plasmon resonance. Biosens. Bioelectron., 142, 111505(2019).
[15] Y. Liu, W. Peng. Fiber-optic surface plasmon resonance sensors and biochemical applications: a review. J. Lightwave Technol., 39, 3781-3791(2021).
[16] C. Caucheteur, T. Guo, J. Albert. Review of plasmonic fiber optic biochemical sensors: improving the limit of detection. Anal. Bioanal. Chem., 407, 3883-3897(2015).
[17] B. D. Gupta, R. Kant. Recent advances in surface plasmon resonance based fiber optic chemical and biosensors utilizing bulk and nanostructures. Opt. Laser Technol., 101, 144-161(2018).
[18] Q. L. Duan, Y. N. Liu, S. S. Chang, H. Y. Chen, J. H. Chen. Surface plasmonic sensors: sensing mechanism and recent applications. Sensors, 21, 5262(2021).
[19] A. K. Sharma, A. K. Pandey, B. Kaur. A review of advancements (2007–2017) in plasmonics-based optical fiber sensors. Opt. Fiber Technol., 43, 20-34(2018).
[20] M. Chauhan, V. K. Singh. Review on recent experimental SPR/LSPR based fiber optic analyte sensors. Opt. Fiber Technol., 64, 102580(2021).
[21] X. D. Wang, O. S. Wolfbeis. Fiber-optic chemical sensors and biosensors (2015–2019). Anal. Chem., 92, 397-430(2020).
[22] M. S. Soares, M. Vidal, N. F. Santos, F. M. Costa, C. Marques, S. O. Pereira, C. Leitao. Immunosensing based on optical fiber technology: recent advances. Biosensors, 11, 305(2021).
[23] J. S. Seok, H. Ju. Plasmonic optical biosensors for detecting C-reactive protein: a review. Micromachines, 11, 895(2020).
[24] M. A. Butt, S. N. Khonina, N. L. Kazanskiy. Plasmonics: a necessity in the field of sensing: a review. Fiber Integr. Opt., 40, 14-47(2021).
[25] S. Lee, H. Song, H. Ahn, S. Kim, J. R. Choi, K. Kim. Fiber-optic localized surface plasmon resonance sensors based on nanomaterials. Sensors, 21, 819(2021).
[26] A. Guerreiro, D. F. Santos, J. M. Baptista. New trends in the simulation of nanosplasmonic optical D-type fiber sensors. Sensors, 19, 1772(2019).
[27] M. Qi, N. M. Y. Zhang, K. W. Li, S. C. Tjin, L. Wei. Hybrid plasmonic fiber-optic sensors. Sensors, 20, 3266(2020).
[28] M. E. Martinez-Hernandez, P. J. Rivero, J. Goicoechea, F. J. Arregui. Trends in the implementation of advanced plasmonic materials in optical fiber sensors (2010–2020). Chemosensors, 9, 64(2021).
[29] B. D. Gupta, A. M. Shrivastav, S. P. Usha. Surface plasmon resonance-based fiber optic sensors utilizing molecular imprinting. Sensors, 16, 1381(2016).
[30] T. Yang, X. L. He, X. Zhou, Z. Y. Lei, Y. L. Wang, J. Yang, D. Cai, S. L. Chen, X. D. Wang. Surface plasmon cavities on optical fiber end-facets for biomolecule and ultrasound detection. Opt. Laser Technol., 101, 468-478(2018).
[31] B. Dastmalchi, P. Tassin, T. Koschny, C. M. Soukoulis. A new perspective on plasmonics: confinement and propagation length of surface plasmons for different materials and geometries. Adv. Opt. Mater., 4, 177-184(2016).
[32] S. Shi, L. B. Wang, R. X. Su, B. S. Liu, R. L. Huang, W. Qi, Z. M. He. A polydopamine-modified optical fiber SPR biosensor using electroless-plated gold films for immunoassays. Biosens. Bioelectron., 74, 454-460(2015).
[33] J. Y. Jing, Q. Wang, B. T. Wang. Refractive index sensing characteristics of carbon nanotube-deposited photonic crystal fiber SPR sensor. Opt. Fiber Technol., 43, 137-144(2018).
[34] X. Zhao, X. Zhang, X. S. Zhu, Y. W. Shi. Long-range surface plasmon resonance sensor based on the GK570/Ag coated hollow fiber with an asymmetric layer structure. Opt. Express, 27, 9550-9560(2019).
[35] X. Zhang, X. S. Zhu, Y. W. Shi. Fiber optic surface plasmon resonance sensor based on a silver-coated large-core suspended-core fiber. Opt. Lett., 44, 4550-4553(2019).
[36] F. Esposito, L. Sansone, A. Srivastava, F. Baldini, S. Campopiano, F. Chiavaioli, M. Giordano, A. Giannetti, A. Iadicicco. Long period grating in double cladding fiber coated with graphene oxide as high-performance optical platform for biosensing. Biosens. Bioelectron., 172, 112747(2021).
[37] P. Urbancova, M. Goraus, D. Pudis, P. Hlubina, A. Kuzma, D. Jandura, J. Durisova, P. Micek. 2D polymer/metal structures for surface plasmon resonance. Appl. Surf. Sci., 530, 147279(2020).
[38] A. Pathak, B. D. Gupta. Palladium nanoparticles embedded PPy shell coated CNTs towards a high performance hydrazine detection through optical fiber plasmonic sensor. Sens. Actuators B, 326, 128717(2021).
[39] S. T. Wang, N. Liu, Q. Cheng, B. Pang, J. T. Lv. Surface plasmon resonance on the antimonene-Fe2O3-copper layer for optical attenuated total reflection spectroscopic application. Plasmonics, 16, 559-566(2020).
[40] C. W. Cheng, S. S. Raja, C. W. Chang, X. Q. Zhang, P. Y. Liu, Y. H. Lee, C. K. Shih, S. Gwo. Epitaxial aluminum plasmonics covering full visible spectrum. Nanophotonics, 10, 627-637(2021).
[41] M. Kanso, S. Cuenot, G. Louarn. Sensitivity of optical fiber sensor based on surface plasmon resonance: modeling and experiments. Plasmonics, 3, 49-57(2008).
[42] P. K. Maharana, R. Jha, S. Palei. Sensitivity enhancement by air mediated graphene multilayer based surface plasmon resonance biosensor for near infrared. Sens. Actuators B, 190, 494-501(2014).
[43] F. C. Chien, S. J. Chen. A sensitivity comparison of optical biosensors based on four different surface plasmon resonance modes. Biosens. Bioelectron., 20, 633-642(2004).
[44] Y. S. Dwivedi, A. K. Sharma, B. D. Gupta. Influence of design parameters on the performance of a surface plasmon sensor based fiber optic sensor. Plasmonics, 3, 79-86(2008).
[45] M. M. Rahman, M. M. Rana, M. S. Rahman, M. S. Anower, M. A. Mollah, A. K. Paul. Sensitivity enhancement of SPR biosensors employing heterostructure of PtSe2 and 2D materials. Opt. Mater., 107, 110123(2020).
[46] H. Agrawal, A. M. Shrivastav, B. D. Gupta. Surface plasmon resonance based optical fiber sensor for atrazine detection using molecular imprinting technique. Sens. Actuators B, 227, 204-211(2016).
[47] F. Chiavaioli, C. A. J. Gouveia, P. A. S. Jorge, F. Baldini. Towards a uniform metrological assessment of grating-based optical fiber sensors: from refractometers to biosensors. Biosensors, 7, 23(2017).
[48] H. P. Loock, P. D. Wentzell. Detection limits of chemical sensors: applications and misapplications. Sens. Actuators B, 173, 157-163(2012).
[49] R. Ravikumar, L. H. Chen, P. Jayaraman, C. L. Poh, C. C. Chan. Chitosan-nickel film based interferometric optical fiber sensor for label-free detection of histidine tagged proteins. Biosens. Bioelectron., 99, 578-585(2018).
[50] C. Liu, Q. Cai, B. J. Xu, W. D. Zhu, L. Zhang, J. L. Zhao, X. F. Chen. Graphene oxide functionalized long period grating for ultrasensitive label-free immunosensing. Biosens. Bioelectron., 94, 200-206(2017).
[51] Z. Cheng, Q. Wang, A. S. Zhu, F. M. Qiu, L. Y. Niu, J. Y. Jing. Au-nanoshells modified surface field enhanced LRSPR biosensor with low LOD for highly sensitive hIgG sensing. Opt. Laser Technol., 134, 106656(2021).
[52] Y. Peng, Y. Zhao, X. G. Hu, Y. Yang. Optical fiber quantum biosensor based on surface plasmon polaritons for the label-free measurement of protein. Sens. Actuators B, 316, 128097(2020).
[53] J. J. Luo, G. S. Liu, W. J. Zhou, S. Q. Hu, L. Chen, Y. F. Chen, Y. H. Luo, Z. Chen. A graphene-PDMS hybrid overcoating enhanced fiber plasmonic temperature sensor with high sensitivity and fast response. J. Mater. Chem. C, 8, 12893-12901(2020).
[54] B. S. Boruah, R. Biswas. In-situ sensing of hazardous heavy metal ions through an ecofriendly scheme. Opt. Laser Technol., 137, 106813(2021).
[55] N. Cennamo, G. D’Agostino, M. Pesavento, L. Zeni. High selectivity and sensitivity sensor based on MIP and SPR in tapered plastic optical fibers for the detection of L-nicotine. Sens. Actuators B, 191, 529-536(2014).
[56] P. H. Zhang, B. Y. Lu, Y. W. Sun, H. X. Yu, K. X. Xu, D. C. Li. Side-polished flexible SPR sensor modified by graphene with
[57] Q. Wang, H. Song, A. S. Zhu, F. M. Qiu. A label-free and anti-interference dual-channel SPR fiber optic sensor with self-compensation for biomarker detection. IEEE Trans. Instrum. Meas., 70, 7002007(2021).
[58] W. H. Tsai, Y. C. Lin, J. K. Tai, Y. C. Tsao. Multi-step structure of side-polished fiber sensor to enhance SPR effect. Opt. Laser Technol., 42, 453-456(2010).
[59] B. Y. Li, Z. C. Sheng, M. Wu, X. Y. Liu, G. Y. Zhou, J. T. Liu, Z. Y. Hou, C. M. Xia. Sensitive real-time monitoring of refractive indices and components using a microstructure optical fiber microfluidic sensor. Opt. Lett., 43, 5070-5073(2018).
[60] I. Dominguez, I. Del Villar, O. Fuentes, J. M. Corres, I. R. Matias. Interdigital concept in photonic sensors based on an array of lossy mode resonances. Sci. Rep., 11, 13228(2021).
[61] G. L. Xiao, Z. T. Ou, H. Y. Yang, Y. P. Xu, J. Y. Chen, H. O. Li, Q. Li, L. Z. Zeng, Y. R. Den, J. Q. Li. An integrated detection based on a multi-parameter plasmonic optical fiber sensor. Sensors, 21, 803(2021).
[62] H. Liu, H. W. Li, Q. Wang, M. Wang, Y. Ding, C. H. Zhu. Simultaneous measurement of temperature and magnetic field based on surface plasmon resonance and Sagnac interference in a D-shaped photonic crystal fiber. Opt. Quant. Electron., 50, 392(2018).
[63] X. C. Yang, Y. Lu, B. L. Liu, J. Q. Yao. Simultaneous measurement of refractive index and temperature based on SPR in D-shaped MOF. Appl. Opt., 56, 4369-4374(2017).
[64] B. Li, F. Zhang, X. Yan, X. N. Zhang, F. Wang, S. G. Li, T. L. Cheng. Numerical analysis of dual-parameter optical fiber sensor with large measurement range based on surface plasmon resonance. IEEE Sens. J., 21, 10719-10725(2021).
[65] T. Ayupova, M. Shaimerdenova, S. Korganbayev, M. Sypabekova, A. Bekmurzayeva, W. Blanc, S. Sales, T. Guo, C. Molardi, D. Tosi. Fiber optic refractive index distributed multi-sensors by scattering-level multiplexing with MgO nanoparticle-doped fibers. IEEE Sens. J., 20, 2504-2510(2020).
[66] M. D. Alonso-Murias, J. S. Velazquez-Gonzalez, D. Monzon-Hernandez. SPR fiber tip sensor for the simultaneous measurement of refractive index, temperature, and level of a liquid. J. Lightwave Technol., 37, 4808-4814(2019).
[67] Q. Wang, J. Y. Jing, X. Z. Wang, L. Y. Niu, W. M. Zhao. A D-shaped fiber long-range surface plasmon resonance sensor with high
[68] H. I. Muri, A. Bano, D. R. Hjelme. LSPR and interferometric sensor modalities combined using a double-clad optical fiber. Sensors, 18, 187(2018).
[69] T. S. Li, L. Q. Zhu, X. C. Yang, X. P. Lou, L. D. Yu. A refractive index sensor based on H-shaped photonic crystal fibers coated with Ag-graphene layers. Sensors, 20, 741(2020).
[70] Z. J. Zhang, Y. Y. Chen, H. J. Liu, H. D. Bae, D. A. Olson, A. K. Gupta, M. Yu. On-fiber plasmonic interferometer for multi-parameter sensing. Opt. Express, 23, 10732-10740(2015).
[71] Y. Liu, Q. Xia, A. Zhou, X. B. Wang, L. B. Yuan. Multi-parameter sensing based on surface plasma resonance with tungsten disulfide sheets coated. Opt. Express, 28, 6084-6094(2020).
[72] S. J. Weng, L. Pei, C. Liu, J. S. Wang, J. Li, T. G. Ning. Double-side polished fiber SPR sensor for simultaneous temperature and refractive index measurement. IEEE Photon. Technol. Lett., 28, 1916-1919(2016).
[73] Y. Yanase, A. Araki, H. Suzuki, T. Tsutsui, T. Kimura, K. Okamoto, T. Nakatani, T. Hiragun, M. Hide. Development of an optical fiber SPR sensor for living cell activation. Biosens. Bioelectron., 25, 1244-1247(2010).
[74] J. Cao, M. H. Tu, T. Sun, K. T. V. Grattan. Wavelength-based localized surface plasmon resonance optical fiber biosensor. Sens. Actuators B, 181, 611-619(2013).
[75] Q. Wang, X. Z. Wang, H. Song, W. M. Zhao, J. Y. Jing. A dual channel self-compensation optical fiber biosensor based on coupling of surface plasmon polariton. Opt. Laser Technol., 124, 106002(2020).
[76] S. Isaacs, I. Abdulhalim. Long range surface plasmon resonance with ultra-high penetration depth for self-referenced sensing and ultra-low detection limit using diverging beam approach. Appl. Phys. Lett., 106, 193701(2015).
[77] M. Vala, S. Etheridge, J. A. Roach, J. Homola. Long-range surface plasmons for sensitive detection of bacterial analytes. Sens. Actuators B, 139, 59-63(2009).
[78] H. Zhang, Y. F. Chen, X. J. Feng, X. Xiong, S. Q. Hu, Z. P. Jiang, J. L. Dong, W. G. Zhu, W. T. Qiu, H. Y. Guan, H. H. Lu, J. H. Yu, Y. C. Zhong, J. Zhang, M. He, Y. H. Luo, Z. Chen. Long-range surface plasmon resonance sensor based on side-polished fiber for biosensing applications. IEEE J. Sel. Top. Quantum Electron., 25, 7101909(2019).
[79] S. Jain, A. Paliwal, V. Gupta, M. Tomar. Refractive index tuning of SiO2 for long range surface plasmon resonance based biosensor. Biosens. Bioelectron., 168, 112508(2020).
[80] K. Liu, M. Xue, J. F. Jiang, T. Wang, P. X. Chang, T. G. Liu. Theoretical modeling of a coupled plasmon waveguide resonance sensor based on multimode optical fiber. Opt. Commun., 410, 552-558(2018).
[81] J. Y. Ma, K. Liu, J. F. Jiang, T. H. Xu, S. Wang, P. X. Chang, Z. Zhang, J. H. Zhang, T. G. Liu. Theoretical and experimental investigation of an all-fiber waveguide coupled surface plasmon resonance sensor with Au-ZnO-Au sandwich structure. IEEE Access, 7, 169961(2019).
[82] K. Zhang, C. G. Du, J. C. Gao. Long-range surface plasmon polariton enhancement in double-electrode structure. Acta Phys. Sinica, 66, 227302(2017).
[83] A. K. Mishra, S. K. Mishra, R. K. Verma. Doped single-wall carbon nanotubes in propagating surface plasmon resonance-based fiber optic refractive index sensing. Plasmonics, 12, 1657-1663(2017).
[84] C. Y. Lin, S. J. Chen. Design of highly sensitive guided-wave surface plasmon resonance biosensor with deep dip using genetic algorithm. Opt. Commun., 445, 155-160(2019).
[85] V. P. Prakashan, G. George, M. S. Sanu, M. S. Sajna, A. C. Saritha, C. Sudarsanakumar, P. R. Biju, C. Joseph, N. V. Unnikrishnan. Investigations on SPR induced Cu@Ag core shell doped SiO2-TiO2-ZrO2 fiber optic sensor for mercury detection. Appl. Surf. Sci., 507, 144957(2020).
[86] K. X. Dong, Y. P. Ji, J. J. Mi, X. T. Zhao, B. Wu, W. X. Huang, J. P. Shi. High sensitivity SPR sensor for liquid phase sample with Ag/PbS/graphene hybrid nanostructure. Opto-Electron. Eng., 44, 198-201(2017).
[87] X. Y. Xuan, S. P. Xu, Y. Liu, H. B. Li, W. Q. Xu, J. R. Lombardi. A long-range surface plasmon resonance/probe/silver nanoparticle (LRSPR-P-NP) nanoantenna configuration for surface-enhanced Raman scattering. J. Phys. Chem. Lett., 3, 2773-2778(2012).
[88] C. T. Yang, R. Mejard, H. J. Griesser, P. O. Bagnaninchi, B. Thierry. Cellular micromotion monitored by long-range surface plasmon resonance with optical fluctuation analysis. Anal. Chem., 87, 1456-1461(2015).
[89] A. W. Wark, H. J. Lee, R. M. Corn. Long-range surface plasmon resonance imaging for bioaffinity sensors. Anal. Chem., 77, 3904-3907(2005).
[90] Y. Y. Huang, M. F. Ding, T. Guo, D. J. Hu, Y. Y. Cao, L. Jin, B. O. Guan. A fiber-optic sensor for neurotransmitters with ultralow concentration: near-infrared plasmonic electromagnetic field enhancement using raspberry-like meso-SiO2 nanospheres. Nanoscale, 9, 14929-14936(2017).
[91] H. Zhang, Y. Sun, S. Gao, H. Q. Zhang, J. Zhang, Y. Bai, D. Q. Song. Studies of gold nanorod-iron oxide nanohybrids for immunoassay based on SPR biosensor. Talanta, 125, 29-35(2014).
[92] S. Shi, L. B. Wang, A. K. Wang, R. L. Huang, L. Ding, R. X. Su, W. Qi, Z. M. He. Bioinspired fabrication of optical fiber SPR sensors for immunoassays using polydopamine accelerated electroless plating. J. Mater. Chem. C, 4, 7554-7562(2016).
[93] Q. Wu, Y. Sun, D. Zhang, S. Li, Y. Zhang, P. Y. Ma, Y. Yu, X. H. Wang, D. Q. Song. Ultrasensitive magnetic field-assisted surface plasmon resonance immunoassay for human cardiac troponin I. Biosens. Bioelectron., 96, 288-293(2017).
[94] S. Li, Q. Wu, P. Y. Ma, Y. Zhang, D. Q. Song, X. H. Wang, Y. Sun. A sensitive SPR biosensor based on hollow gold nanospheres and improved sandwich assay with PDA-Ag@Fe3O4/rGO. Talanta, 180, 156-161(2018).
[95] C. Fenzl, T. Hirsch, A. J. Baeumner. Liposomes with high refractive index encapsulants as tunable signal amplification tools in surface plasmon resonance spectroscopy. Anal. Chem., 87, 11157-11163(2015).
[96] H. Komatsu, M. Miyachi, E. Fujii, D. Citterio, K. Yamada, Y. Sato, K. Kurihara, H. Kawaguchi, K. Suzuki. SPR sensor signal amplification based on dye-doped polymer particles. Sci. Technol. Adv. Mater., 7, 150-155(2006).
[97] X. H. Zhu, Y. Y. Zhang, M. L. Liu, Y. Liu. 2D titanium carbide MXenes as emerging optical biosensing platforms. Biosens. Bioelectron., 171, 112730(2021).
[98] L. Y. Niu, Q. Wang, J. Y. Jing, W. M. Zhao. Sensitivity enhanced D-type large-core fiber SPR sensor based on Gold nanoparticle/Au film co-modification. Opt. Commun., 450, 287-295(2019).
[99] H. Z. Yuan, W. Ji, S. W. Chu, S. Y. Qian, F. Wang, J. F. Masson, X. Y. Han, W. Peng. Fiber-optic surface plasmon resonance glucose sensor enhanced with phenylboronic acid modified Au nanoparticles. Biosens. Bioelectron., 117, 637-643(2018).
[100] K. Liu, J. H. Zhang, J. F. Jiang, T. H. Xu, S. Wang, P. X. Chang, Z. Zhang, J. Y. Ma, T. G. Liu. Multi-layer optical fiber surface plasmon resonance biosensor based on a sandwich structure of polydopamine-MoSe2@Au nanoparticles-polydopamine. Biomed. Opt. Express, 11, 6840-6851(2020).
[101] A. M. Shrivastav, S. P. Usha, B. D. Gupta. Highly sensitive and selective erythromycin nanosensor employing fiber optic SPR/ERY imprinted nanostructure: application in milk and honey. Biosens. Bioelectron., 90, 516-524(2017).
[102] H. Song, H. X. Zhang, Z. Sun, Z. Y. Ren, X. Y. Yang, Q. Wang. Triangular silver nanoparticle U-bent fiber sensor based on localized surface plasmon resonance. AIP Adv., 9, 085307(2019).
[103] M. Sun, Y. X. Wang, Z. N. Chen, Y. D. Gong, J. L. Lim, X. M. Qing. Nanostars on a fiber facet with near field enhancement for surface-enhanced Raman scattering detection. Appl. Phys. A, 115, 87-91(2014).
[104] F. Xia, H. Song, Y. Zhao, W. M. Zhao, Q. Wang, X. Z. Wang, B. T. Wang, Z. X. Dai. Ultra-high sensitivity SPR fiber sensor based on multilayer nanoparticle and Au film coupling enhancement. Measurement, 164, 108083(2020).
[105] D. J. Feng, G. X. Liu, Q. Li, J. Cui, J. Zheng, Z. C. Ye. Design of infrared SPR sensor based on bimetallic nanowire gratings on plastic optical fiber surface. IEEE Sens. J., 15, 255-259(2015).
[106] R. Kant, R. Tabassum, B. D. Gupta. Xanthine oxidase functionalized Ta2O5 nanostructures as a novel scaffold for highly sensitive SPR based fiber optic xanthine sensor. Biosens. Bioelectron., 99, 637-645(2018).
[107] S. Parveen, A. Pathak, B. D. Gupta. Fiber optic SPR nanosensor based on synergistic effects of CNT/Cu-nanoparticles composite for ultratrace sensing of nitrate. Sens. Actuators B, 246, 910-919(2017).
[108] X. Jiang, Q. Wang. Refractive index sensitivity enhancement of optical fiber SPR sensor utilizing layer of MWCNT/PtNPs composite. Opt. Fiber Technol., 51, 118-124(2019).
[109] A. V. Kabashin, P. Evans, S. Pastkovsky, W. Hendren, G. A. Wurtz, R. Atkinson, R. Pollard, V. A. Podolskiy, A. V. Zayats. Plasmonic nanorod metamaterials for biosensing. Nat. Mater., 8, 867-871(2009).
[110] Y. N. Zhang, B. R. Tao, Q. L. Wu, B. Han. Reflective SPR sensor for simultaneous measurement of nitrate concentration and temperature. IEEE Trans. Instrum. Meas., 68, 4566-4573(2019).
[111] A. Pathak, S. Parveen, B. D. Gupta. Fibre optic SPR sensor using functionalized CNTs for the detection of SMX: comparison with enzymatic approach. Plasmonics, 13, 189-202(2018).
[112] A. Pathak, B. D. Gupta. Ultra-selective fiber optic SPR platform for the sensing of dopamine in synthetic cerebrospinal fluid incorporating permselective nafion membrane and surface imprinted MWCNTs-PPy matrix. Biosens. Bioelectron., 133, 205-214(2019).
[113] S. K. Mishra, S. N. Tripathi, V. Choudhary, B. D. Gupta. Surface plasmon resonance-based fiber optic methane gas sensor utilizing graphene-carbon nanotubes-poly(methyl methacrylate) hybrid nanocomposite. Plasmonics, 10, 1147-1157(2015).
[114] R. Tabassum, B. D. Gupta. Simultaneous tuning of electric field intensity and structural properties of ZnO: graphene nanostructures for FOSPR based nicotine sensor. Biosens. Bioelectron., 91, 762-769(2017).
[115] B. T. Wang, Y. X. Niu, S. W. Zheng, Y. H. Yin, M. Ding. An optical fiber immunosensor with a low detection limit based on plasmon coupling enhancement. J. Lightwave Technol., 38, 3781-3788(2020).
[116] W. Yang, J. Yu, X. T. Xi, Y. Sun, Y. M. Shen, W. W. Yue, C. Zhang, S. Z. Jiang. Preparation of graphene/ITO nanorod metamaterial/U-bent-annealing fiber sensor and DNA biomolecule detection. Nanomaterials, 9, 1154(2019).
[117] A. K. Pathak, V. K. Singh. SPR based optical fiber refractive index sensor using silver nanowire assisted CSMFC. IEEE Photon. Technol. Lett., 32, 465-468(2020).
[118] Y. Guo, J. S. Li, S. G. Li, Y. D. Liu, S. H. Zhang, J. Wang, S. Wang, W. X. Zhang, T. L. Cheng, R. Hao. Dual-polarized optical sensing of microstructure fiber with pentagonal-lattice based on surface plasmon resonance in the near-IR spectrum. Optik, 202, 163671(2020).
[119] Q. Wu, N. B. Li, Y. Wang, Y. C. Xu, J. D. Wu, G. R. Jia, F. J. Ji, X. D. Fang, F. F. Chen, X. Q. Cui. Ultrasensitive and selective determination of carcinoembryonic antigen using multifunctional ultrathin amino-functionalized Ti3C2-MXene nanosheets. Anal. Chem., 92, 3354-3360(2020).
[120] L. X. Kang, C. M. Das, D. Liu, M. W. Chen, P. Coquet, G. Hong, K. T. Yong. A comparative performance evaluation of 2D nanomaterials for applications in plasmonic biosensing. Phys. Status Solidi A, 217, 2000255(2020).
[121] N. Li, J. J. Guo, Y. W. Ding, Y. Q. Hu, C. H. Zhao, C. J. Zhao. Direct regulation of double cation defects at the A1A2 site for a high-performance oxygen evolution reaction perovskite catalyst. ACS Appl. Mater. Interfaces, 13, 332-340(2021).
[122] D. T. Nurrohman, Y. H. Wang, N. F. Chiu. Exploring graphene and MoS2 chips based surface plasmon resonance biosensors for diagnostic applications. Front. Chem., 8, 728(2020).
[123] R. Kumar, S. Pal, A. Verma, Y. K. Prajapati, J. P. Saini. Effect of silicon on sensitivity of SPR biosensor using hybrid nanostructure of black phosphorus and MXene. Superlattices Microstruct., 145, 106591(2020).
[124] Y. F. Yuan, X. T. Yu, Q. L. Ouyang, Y. H. Shao, J. Song, J. L. Qu, K. T. Yong. Highly anisotropic black phosphorous-graphene hybrid architecture for ultrassensitive plasmonic biosensing: theoretical insight. 2D Mater., 5, 025015(2018).
[125] H. Song, Q. Wang, W. M. Zhao. A novel SPR sensor sensitivity-enhancing method for immunoassay by inserting MoS2 nanosheets between metal film and fiber. Opt. Laser Eng., 132, 106135(2020).
[126] S. Pal, A. Verma, Y. K. Prajapati, J. P. Saini. Sensitive detection using heterostructure of black phosphorus, transition metal di-chalcogenides and MXene in SPR sensor. Appl. Phys. A, 126, 809(2020).
[127] M. S. Rahman, M. S. Anower, L. F. Abdulrazak. Utilization of a phosphorene-graphene/TMDC heterostructure in a surface plasmon resonance-based fiber optic biosensor. Photon. Nanostr. Fundam. Appl., 35, 100711(2019).
[128] Y. Z. Chen, Y. Q. Ge, W. C. Huang, Z. J. Li, L. M. Wu, H. Zhang, X. J. Li. Refractive index sensors based on Ti3C2T
[129] Z. C. Xia, F. H. Chu, Z. L. Bian, Z. Zhang, J. L. Li, Z. Guo. Study of surface plasmon resonance sensor based on polymer-tipped optical fiber with barium titanate layer. J. Lightwave Technol., 38, 912-918(2020).
[130] Y. Vasimalla, H. S. Pradhan, R. J. Pandya. SPR performance enhancement for DNA hybridization employing black phosphorus, silver, and silicon. Appl. Opt., 59, 7299-7307(2020).
[131] Q. Wu, N. B. Li, Y. Wang, Y. Liu, Y. C. Xu, S. T. Wei, J. D. Wu, G. R. Jia, X. D. Fang, F. F. Chen, X. Q. Cui. A 2D transition metal carbide MXene-based SPR biosensor for ultrasensitive carcinoembryonic antigen detection. Biosens. Bioelectron., 144, 111697(2019).
[132] N. Mudgal, P. Yupapin, J. Ali, G. Singh. BaTiO3-graphene-affinity layer-based surface plasmon resonance (SPR) biosensor for pseudomonas bacterial detection. Plasmonics, 15, 1221-1229(2020).
[133] A. Srivastava, R. Das, Y. K. Prajapati. Effect of perovskite material on performance of surface plasmon resonance biosensor. IET Optoelectron., 14, 256-265(2020).
[134] M. Alagdar, B. Yousif, N. F. Areed, M. Elzalabani. Highly sensitive fiber optic surface plasmon resonance sensor employing 2D nanomaterials. Appl. Phys. A, 126, 522(2020).
[135] , M. K. Yadav, P. Kumar, R. K. Verma. Detection of adulteration in pure honey utilizing Ag-graphene oxide coated fiber optic SPR probes. Food Chem., 332, 127346(2020).
[136] Q. Wang, X. Jiang, L. Y. Niu, X. C. Fan. Enhanced sensitivity of bimetallic optical fiber SPR sensor based on MoS2 nanosheets. Opt. Laser Eng., 128, 105997(2020).
[137] S. Kaushik, U. K. Tiwari, A. Deep, R. K. Sinha. Two-dimensional transition metal dichalcogenides assisted biofunctionalized optical fiber SPR biosensor for efficient and rapid detection of bovine serum albumin. Sci. Rep., 9, 6987(2019).
[138] K. Liu, J. H. Zhang, J. F. Jiang, T. H. Xu, S. Wang, P. X. Chang, Z. Zhang, J. Y. Ma, T. G. Liu. MoSe2-Au Based sensitivity enhanced optical fiber surface plasmon resonance biosensor for detection of goat-anti-rabbit IgG. IEEE Access, 8, 660-668(2020).
[139] A. K. Mishra, S. K. Mishra, R. K. Verma. Graphene and beyond graphene MoS2: a new window in surface-plasmon-resonance-based fiber optic sensing. J. Phys. Chem. C, 120, 2893-2900(2016).
[140] A. K. Sharma, A. K. Pandey, B. Kaur. Simulation study on comprehensive sensing enhancement of BlueP/MoS2-and BlueP/WS2-based fluoride fiber surface plasmon resonance sensors: analysis founded on damping, field, and optical power. Appl. Opt., 58, 4518-4525(2019).
[141] M. S. Rahman, M. S. Anower, L. F. Abdulrazak. Modeling of a fiber optic SPR biosensor employing tin selenide (SnSe) allotropes. Results Phys., 15, 102623(2019).
[142] S. Sharma, B. D. Gupta. Surface plasmon resonance based highly selective fiber optic dopamine sensor fabricated using molecular imprinted GNP/SnO2 nanocomposite. J. Lightwave Technol., 36, 5956-5962(2018).
[143] R. Kant, B. D. Gupta. Fiber-optic SPR based acetylcholine biosensor using enzyme functionalized Ta2O5 nanoflakes for Alzheimer’s disease diagnosis. J. Lightwave Technol., 36, 4018-4024(2018).
[144] , R. K. Verma. On the application of few layer Ti3C2 MXene on fiber optic SPR sensor for performance enhancement. Eur. Phys. J. D, 75, 5(2021).
[145] Q. Wang, L. Y. Niu, J. Y. Jing, W. M. Zhao. Barium titanate film based fiber optic surface plasmon sensor with high sensitivity. Opt. Laser Technol., 124, 105899(2020).
[146] S. H. Sun, C. B. Murray, D. Weller, L. Folks, A. Moser. Monodisperse FePt nanoparticles and ferromagnetic FePt nanocrystal superlattices. Science, 287, 1989-1992(2000).
[147] N. A. Sakthivel, A. Dass. Aromatic thiolate-protected series of gold nanomolecules and a contrary structural trend in size evolution. Acc. Chem. Res., 51, 1774-1783(2018).
[148] H. Wu, F. Bai, Z. C. Sun, R. E. Haddad, D. M. Boye, Z. W. Wang, J. Y. Huang, H. Y. Fan. Nanostructured gold architectures formed through high pressure-driven sintering of spherical nanoparticle arrays. J. Am. Chem. Soc., 132, 12826-12828(2010).
[149] J. J. Cheng, G. Le Saux, J. Gao, T. Buffeteau, Y. Battie, P. Barois, V. Ponsinet, M. H. Delville, O. Ersen, E. Pouget, R. Oda. GoldHelix: gold nanoparticles forming 3D helical superstructures with controlled morphology and strong chiroptical property. ACS Nano, 11, 3806-3818(2017).
[150] C. Li, Z. Li, S. L. Li, Y. N. Zhang, B. P. Sun, Y. H. Yu, H. Y. Ren, S. Z. Jiang, W. W. Yue. LSPR optical fiber biosensor based on a 3D composite structure of gold nanoparticles and multilayer graphene films. Opt. Express, 28, 6071-6083(2020).
[151] D. Daems, W. Pfeifer, I. Rutten, B. Sacca, D. Spasic, J. Lammertyn. Three-dimensional DNA origami as programmable anchoring points for bioreceptors in fiber optic surface plasmon resonance biosensing. ACS Appl. Mater. Interfaces, 10, 23539-23547(2018).
[152] Y. Zhao, M. Lei, S. X. Liu, Q. Zhao. Smart hydrogel-based optical fiber SPR sensor for pH measurements. Sens. Actuators B, 261, 226-232(2018).
[153] L. X. Kong, Y. X. Zhang, W. G. Zhang, Y. S. Zhang, T. Y. Yan, P. C. Geng, B. Wang. Lab-on-tip: protruding-shaped all-fiber plasmonic microtip probe toward
[154] S. J. Guo, S. J. Dong. Metal nanomaterial-based self-assembly: development, electrochemical sensing and SERS applications. J. Mater. Chem., 21, 16704-16716(2011).
[155] P. Colson, C. Henrist, R. Cloots. Nanosphere lithography: a powerful method for the controlled manufacturing of nanomaterials. J. Nanomater., 2013, 948510(2013).
[156] Y. Wang, Z. J. Cui, X. J. Zhang, X. Zhang, Y. Q. Zhu, S. G. Chen, H. Hu. Excitation of surface plasmon resonance on multiwalled carbon nanotube metasurfaces for pesticide sensors. ACS Appl. Mater. Interfaces, 12, 52082-52088(2020).
[157] Q. Wang, L. Wang. Lab-on-fiber: plasmonic nano-arrays for sensing. Nanoscale, 12, 7485-7499(2020).
[158] V. G. Kravets, A. V. Kabashin, W. L. Barnes, A. N. Grigorenko. Plasmonic surface lattice resonances: a review of properties and applications. Chem. Rev., 118, 5912-5951(2018).
[159] X. L. He, H. Yi, J. Long, X. Zhou, J. Yang, T. Yang. Plasmonic crystal cavity on single-mode optical fiber end facet for label-free biosensing. Appl. Phys. Lett., 108, 231105(2016).
[160] I. Antohe, D. Spasic, F. Delport, J. Q. Li, J. Lammertyn. Nanoscale patterning of gold-coated optical fibers for improved plasmonic sensing. Nanotechnology, 28, 215301(2017).
[161] N. Wang, M. Zeisberger, U. Hubner, M. A. Schmidt. Nanotrimer enhanced optical fiber tips implemented by electron beam lithography. Opt. Mater. Express, 8, 2246-2255(2018).
[162] C. Ciraci, R. T. Hill, J. J. Mock, Y. Urzhumov, A. I. Fernandez-Dominguez, S. A. Maier, J. B. Pendry, A. Chilkoti, D. R. Smith. Probing the ultimate limits of plasmonic enhancement. Science, 337, 1072-1074(2012).
[163] W. Q. Zhu, R. Esteban, A. G. Borisov, J. J. Baumberg, P. Nordlander, H. J. Lezec, J. Aizpurua, K. B. Crozier. Quantum mechanical effects in plasmonic structures with subnanometre gaps. Nat. Commun., 7, 11495(2016).
[164] J. J. Baumberg, J. Aizpurua, M. H. Mikkelsen, D. R. Smith. Extreme nanophotonics from ultrathin metallic gaps. Nat. Mater., 18, 668-678(2019).
[165] C. Lee, B. Lawrie, R. Pooser, K. G. Lee, C. Rockstuhl, M. Tame. Quantum plasmonic sensors. Chem. Rev., 121, 4743-4804(2021).
[166] S. Kim, N. Yu, X. Z. Ma, Y. Z. Zhu, Q. S. Liu, M. Liu, R. X. Yan. High external-efficiency nanofocusing for lens-free near-field optical nanoscopy. Nat. Photonics, 13, 636-643(2019).
[167] M. Danckwerts, L. Novotny. Optical frequency mixing at coupled gold nanoparticles. Phys. Rev. Lett., 98, 026104(2007).
[168] P. Anger, P. Bharadwaj, L. Novotny. Enhancement and quenching of single-molecule fluorescence. Phys. Rev. Lett., 96, 113002(2006).
[169] X. L. He, L. Yang, T. Yang. Optical nanofocusing by tapering coupled photonic-plasmonic waveguides. Opt. Express, 19, 12865-12872(2011).
[170] C. M. Miyazaki, D. J. Kinahan, R. Mishra, F. Mangwanya, N. Kilcawley, M. Ferreira, J. Ducree. Label-free, spatially multiplexed SPR detection of immunoassays on a highly integrated centrifugal lab-on-a-disc platform. Biosens. Bioelectron., 119, 86-93(2018).
[171] M. Q. Zou, C. R. Liao, S. Liu, C. Xiong, C. Zhao, J. L. Zhao, Z. S. Gan, Y. P. Chen, K. M. Yang, D. Liu, Y. Wang, Y. P. Wang. Fiber-tip polymer clamped-beam probe for high-sensitivity nanoforce measurements. Light Sci. Appl., 10, 171(2021).
[172] T. Q. Zhang, M. D. Wang, Y. Xue, J. L. Xu, Z. D. Xie, S. N. Zhu. Liquid metal as a broadband saturable absorber for passively Q-switched lasers. Chin. Opt. Lett., 18, 111801(2020).
[173] R. T. Liu, X. Y. Ye, T. H. Cui. Recent progress of biomarker detection sensors. Research, 2020, 7949037(2020).
[174] P. X. Chang, K. Liu, J. F. Jiang, T. H. Xu, Z. Zhang, J. Y. Ma, Y. H. Zhao, J. H. Zhang, X. B. Li, T. G. Liu. The temperature responsive mechanism of fiber surface plasmon resonance sensor. Sens. Actuators A Phys., 309, 112022(2020).
[175] L. M. Wu, Z. T. Ling, L. Y. Jiang, J. Guo, X. Y. Dai, Y. J. Xiang, D. Y. Fan. Long-range surface plasmon with graphene for enhancing the sensitivity and detection accuracy of biosensor. IEEE Photon. J., 8, 4801409(2016).
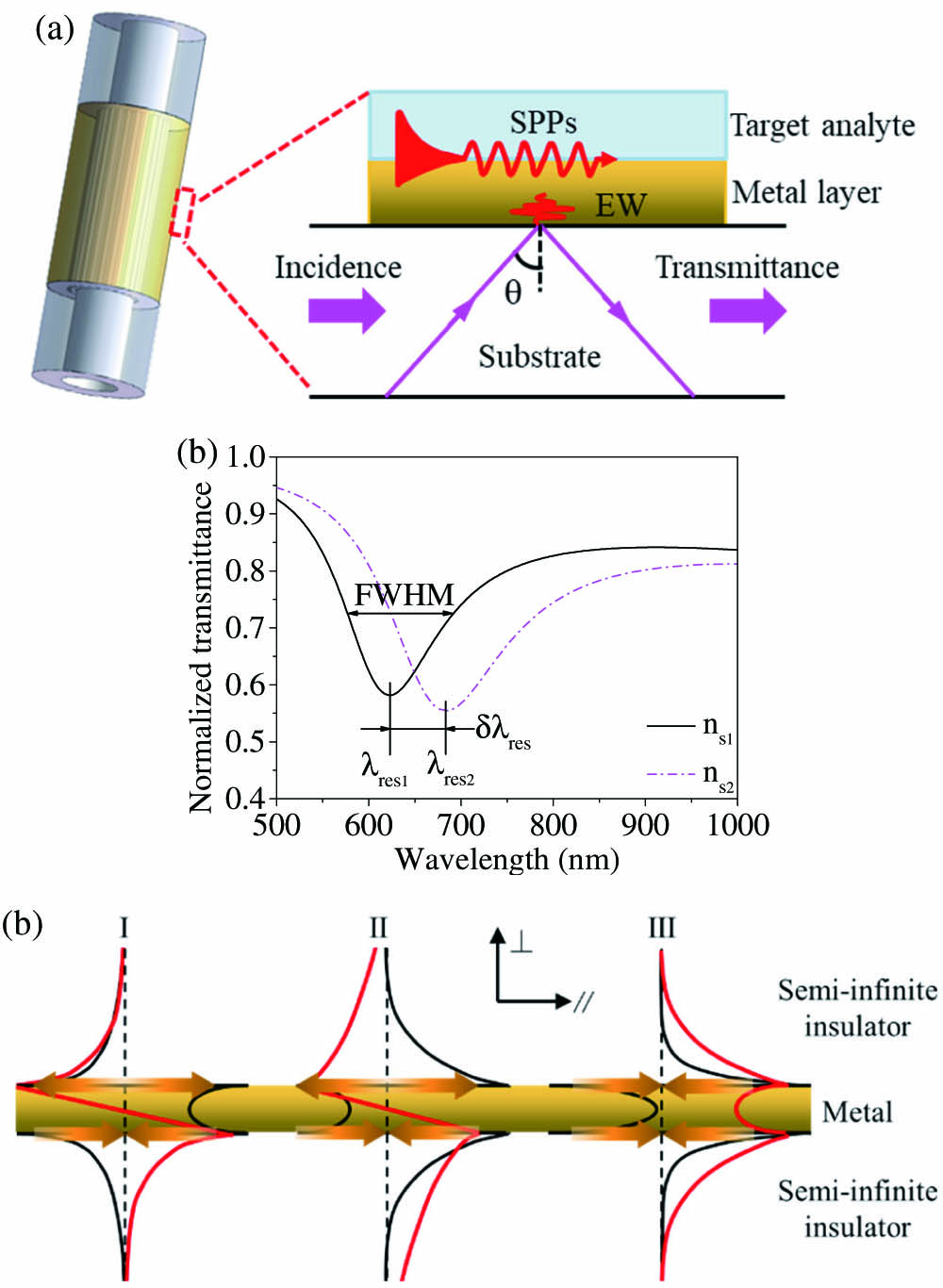
Set citation alerts for the article
Please enter your email address