
- Photonics Research
- Vol. 9, Issue 12, 2511 (2021)
Abstract
1. INTRODUCTION
In recent years, optical phased arrays (OPAs) have gained interest as a solid-state beam steering solution. Similar to the principle of microwave radar, OPAs [1–5] achieve beam steering by adjusting the phases among different waveguides. Compared to traditional mechanical beam steering method, OPAs feature compact size, low power consumption, and low cost [6]. The most attractive feature is that the OPAs can be integrated with CMOS circuits, forming an on-chip optoelectronic system. It is suitable for various applications that need to control beam steering, especially in a light detection and ranging (LIDAR) system. As key component of the LIDAR system, OPAs are expected to realize wide steering range, high resolution, and yet low power consumption and fast modulation speed simultaneously.
To date, the trade-offs between wide angle steering and high resolution exist in the phase control horizontal axis (
The OPAs with nonuniform antenna pitches [13–15] are a promising approach to achieve wide steering range and high resolution simultaneously. Optimized sparse pitches among the waveguides expand the emission aperture effectively, thereby increasing the resolution of
Sign up for Photonics Research TOC. Get the latest issue of Photonics Research delivered right to you!Sign up now
The resolution in the wavelength controlled vertical axis (
Thermo-optic modulation [19,20] is commonly used for phase control of OPAs. However, thermo-optic phase shifters are power hungry and usually consume tens of milliwatts of power. For a large-scale OPA, the overall power consumption can be even as high as several tens of watts [12]. It results in chip heat dissipation problem and thermal cross talk. The 3 dB bandwidth of thermo-optic phase shifter is at kilohertz (kHz) level, and the optical response is usually at microsecond level [21]. On the other hand, the
To solve the mentioned issues, we propose a novel OPA structure incorporated with specifically designed dual-level waveguide grating antennas [17] based on a SiN-on-SOI (silicon-on-insulator) platform [23]. We report two high-performance 128-channel OPAs with fishbone antenna and chain antenna, respectively. Attributed to the nonuniform antenna pitch, only 128 waveguides are used to generate a 4 mm aperture. Through nonuniform antenna pitch distribution and wide-angle antennas, the steering ranges in the
2. STRUCTURE AND CHARACTERISTICS
Figure 1(a) shows the optical microscope image of the fabricated 128-channel OPA. The chip includes a
Figure 1.(a) Optical microscope image of 128-channel OPA. (b) Scanning electron microscope (SEM) image of a fishbone waveguide grating. (c) SEM image of a chain waveguide grating. (d) Transmission electron microscope (TEM) image of dual-level misaligned waveguide grating with the cladding removed. (e) Schematic of a
To achieve high resolution and emission efficiency in
Figure 1(e) shows a schematic diagram of the
The emitting profiles of the chain and the fishbone grating antennas are analyzed. Figure 2(a) shows the 3D finite difference time domain (FDTD) simulation results for the FWHM of the emitting profile as functions of the etch hole width
Figure 2.Simulation results for emitting profiles of the chain and fishbone grating antennas. (a) The plot for FWHM of the emitting profile against etch hole width
As shown in Figs. 2(c) and 2(d), we simulate the far-field steering performance of the chain antenna OPA and fishbone antenna OPA respectively. The average waveguide spacing is 29.7 μm. Because both gratings are apodized gratings, the shown profiles are average of periodic grating profile. In the research of OPA LIDAR, sidelobe suppression ratio (SMSR) is generally about 10 dB (10% of the maximum) [7,13]. In this paper, the steering range is defined as the width of steering envelope when the main beam intensity drops from the maximum to 20%. That is, during the entire steering process, the main beam intensity is at least twice as high as the sidelobe, so that the main lobe signal can be easily distinguished. Comparing the two profiles, it shows that the steering envelope of chain grating is much broader than that of the fishbone. To avoid beam aliasing in the 180° FOV, a nonuniform waveguide pitch strategy is adopted [24]. With nonuniform antenna spacing, chain antenna OPA theoretical steering range is 140° and fishbone antenna OPA is 100°. Compared to the Si-based antenna, the steering ability of the
3. EXPERIMENTS
A. OPA Device Performance
According to the far-field condition [25], far-field beam steering test needs to be performed at a long distance, so the beam steering test was measured by rotating the chip. The beam steering performance of chain and fishbone antenna OPAs in
Figure 3.(a) and (b) Beam steering performance in
The wavelength control steering result is shown in Fig. 4, with a 1350–1630 nm tunable laser, the chain antenna OPA steers to 19.23° and the fishbone antenna OPA steers to 19.4°. The periods of the fishbone and chain grating antenna are all the same, so the steering angle in
Figure 4.Beam steering performance in
Figures 5(a) and 5(b) are the main lobe cross section curves of the chain antenna OPA 15 m away. The theoretical diffraction spot size is
Figure 5.(a) and (b) Main beam cross section measurement 15 m away from the chain antenna OPA in the
As shown in Figs. 5(c) and 5(d), the measured far-field FWHM spot size of the fishbone antenna OPA is
In our previous work, the performances of dual-level chain and fishbone apodized waveguide gratings were studied [17]. The etched region of the fishbone structure is far away from the mode center, so it is robust to process variation. The perturbation of the fishbone grating antenna produces a long and uniform radiation and thus obtains a small divergence in
The large aperture of OPAs facilitates high-resolution scanning. As shown in Fig. 5(e), we steer 37 spots in about 1° in
To show the main beam quality across the FOV of the proposed OPAs, the main beam divergence in the
Figure 6.(a) and (b) Main beam divergence in the
The low main beam power efficiency is mainly from the large on-chip insertion loss and the dense sidelobes in the whole FOV. The loss of each part of the chip includes 1.45 dB in the input
B. FMCW Ranging Performance
Using the proposed OPAs, a frequency-modulated continuous-wave (FMCW) ranging system [26–28] is performed. The schematic of FMCW LIDAR photonic circuit with triangular modulation is illustrated in Fig. 7(a). OPA is added to the system as the transmitter (TX) and receiver (RX) for on-chip beam collimation. A single frequency laser is modulated by single sideband (SSB) modulator to generate frequency modulated signal; the frequency sweep rate is 30 THz/s, and the modulation bandwidth is 3 GHz from 8.5 to 11.5 GHz. Then the signal is amplified by an erbium-doped fiber amplifier (EDFA) and divided into TX signal and local oscillator (LO) signal. The TX signal is amplified again, and then it is coupled into the fishbone antenna OPA for light collimation. The light reflected from the target is received through a collimator and coherently beaten against the LO. A balanced detector containing a transimpedance amplifier (TIA) converts the light signal into voltage signal, and the multichannel high-speed analog-to-digital converter (ADC) reads the voltage signal, which can be analyzed by digital signal process (DSP). Using coherent detection, a time delay between an RX signal and an LO signal will create a beat frequency. Beat frequency is proportional to the target distance. The target distance is given by
Figure 7.(a) Schematic of FMCW LIDAR photonic circuit with triangular modulation. (b) Measured beat frequency at various distances with OPA acting as a TX or RX. The inset is the measured beat frequency at various distances at 5 cm intervals. (c) 116 repeated measurements of beat frequency at 100 m.
Using the FMCW system, up to 100 m distance was measured. Figure 7(b) shows the measured beat frequency at different distances, a 90% reflectivity scatter plate is placed at a known distance to detect the distance, and the target distance ranges from 5 to 100 m. The fishbone antenna OPA is used as the TX. The inset is the measured beat frequency at various distances at 5 cm intervals. The ranging resolution is 5.09 cm. As shown in Fig. 7(c), distance at 100 m was measured 116 times, and the absolute error of ranging accuracy is 3.38 cm. The reflected signal from 100 m distance is detected with 26 dBm input transmitter power. Such a long detection range benefits from the
Figure 8(a) shows the concept of the FMCW LIDAR photonic circuit for long-reach detection. Figures 8(b) and 8(c) illustrate Fourier transform waveform data of the chain antenna OPA and the fishbone antenna OPA steering to 0° separately at about 100 m. The signal-to-noise pedestal ratios (SPNRs) [30] of the chain antenna OPA and the fishbone antenna OPA are 9.15 dB and 13.07 dB. The difference in the SPNR between the chain antenna OPA and the fishbone antenna OPA is mainly because the divergence in
Figure 8.(a) Concept of FMCW LIDAR photonic circuit for long-reach detection. (b) and (c) Fourier transform waveform data of the chain antenna OPA and fishbone antenna OPA steering to 0° separately at about 100 m. The signal-to-noise pedestal ratios of the chain antenna OPA and fishbone antenna OPA are 9.15 dB and 13.07 dB. (d) Measured beat frequency at different distances with the fishbone antenna OPA acting as a TX or RX.
As shown in Fig. 8(d), the beat frequency of the fishbone antenna OPA acting as an RX or TX is almost the same. They prove that the OPA is a promising candidate for transceiving optical signal in coherent detection, attributed to the small divergence, high emission efficiency of the antenna, and low nonlinearity of the
4. DISCUSSION
To demonstrate wide steering range and high resolution of proposed devices, the performances of the devices are compared to selected results from the literature in Table 1. By comparison, the chain antenna OPA has wide steering range in
Performance Comparison of State-of-the-art OPAs
Ref. [ | Ref. [ | Ref. [ | Ref. [ | Ref. [ | This Work (Fishbone) | This Work (Chain) | |
---|---|---|---|---|---|---|---|
Number of channels | 64 | 1024 | 32 | 128 | 8192 | 128 | 128 |
Pitch (μm) | 0.775 | 2 | 1 | 7.246 | 1 | 29.7 | 29.7 |
Horizontal steering (°) | 120 | 45 | 96 | 80 | 100 | 100 | 140 |
Divergence (°) | 1.6 | 0.03 | |||||
Aperture (mm) | 0.0496 | 2.048 |
For nonuniform spacing OPAs, waveguide pitch is average pitch;
m and n are data unknown.
As shown in Table 1, the largest scale OPA has 8192 channels with 1 μm waveguide pitch. The largest aperture enables the smallest divergence in
Further research should be undertaken to investigate how to achieve small divergence and wide steering angle simultaneously. The chain antenna OPA has the widest steering range in
The concentrated main beam obtained by super sparse waveguide pitch compensates for the chip loss, which is beneficial to long-range detecting. Reducing chip loss and maintaining small divergence at the same time should be considered in further study.
5. CONCLUSION
In conclusion, we have demonstrated two 128-channel OPAs with large steering range and small divergence. Compared with the fishbone structure, the chain antenna OPA has a unique advantage in expanding the beam steering range. It achieves 140° steering, which is the widest horizontal steering range for two-dimensional OPAs to our best knowledge. In the phase control horizontal axis, the devices achieve a small divergence by virtue of a 4 mm aperture. In the wavelength control vertical axis, the
References
[1] S. A. Miller, Y. C. Chang, C. T. Phare, C. S. Min, M. Lipson. Large-scale optical phased array using a low-power multi-pass silicon photonic platform. Optica, 7, 3-6(2020).
[2] C. V. Poulton, M. J. Byrd, P. Russo, E. Timurdogan, M. Khandaker, D. Vermeulen, M. R. Watts. Long-range LiDAR and free-space data communication with high-performance optical phased arrays. IEEE J. Sel. Top. Quantum Electron., 25, 7700108(2019).
[3] N. A. Tyler, D. Fowler, S. Malhouitre, S. Garcia, P. Grosse, W. Rabaud, B. Szelag. SiN integrated optical phased arrays for two-dimensional beam steering at a single near-infrared wavelength. Opt. Express, 27, 5851-5858(2019).
[4] Q. Wang, S. Wang, L. Jia, Y. Cai, M. Yu. Silicon nitride assisted 1×64 optical phased array based on SOI platform. Opt. Express, 29, 10509-10517(2021).
[5] L. Zhang, Y. Li, M. Tao, Y. Wang, Y. Hou, B. Chen, Y. Li, L. Qin, F. Gao, X. Luo. Large-scale integrated multi-lines optical phased array chip. IEEE Photon. J., 12, 6601208(2020).
[6] C. P. Hsu, B. Li, B. Solano-Rivas, A. R. Gohil, V. Donzella. A review and perspective on optical phased array for automotive LiDAR. IEEE J. Sel. Top. Quantum Electron., 27, 8300416(2021).
[7] C. T. Phare, C. S. Min, J. Sharma, S. Ahasan, M. Lipson. Silicon optical phased array with grating lobe-free beam formation over 180 degree field of view. CLEO: Science and Innovations, SM3I.2(2018).
[8] W. L. Stutzman, G. A. Thiele. Antenna Theory and Design(2013).
[9] S. J. Orfanidis. Electromagnetic Waves and Antennas(2016).
[10] C. V. Poulton, M. J. Byrd, B. Moss, E. Timurdogan, M. R. Watts. 8192-element optical phased array with 100° steering range and flip-chip CMOS. CLEO: Applications and Technology, JTh4A.3(2020).
[11] C. V. Poulton, M. J. Byrd, M. Raval, S. Zhan, M. R. Watts. Large-scale visible and infrared optical phased arrays in silicon nitride. CLEO: Science & Innovations, STh1M.1(2017).
[12] S. W. Chung, H. Abediasl, H. Hashemi. A monolithically integrated large-scale optical phased array in silicon-on-insulator CMOS. IEEE J. Solid-State Circuits, 53, 275-296(2017).
[13] D. N. Hutchison, S. Jie, J. K. Doylend, R. Kumar, H. Rong. High-resolution aliasing-free optical beam steering. Optica, 3, 887-890(2016).
[14] C. S. Min, A. Mohanty, K. Watson, G. R. Bhatt, M. Lipson. Chip-scale blue light phased array. Opt. Lett., 45, 1934-1937(2020).
[15] R. Fatemi, A. Khachaturian, A. Hajimiri. A nonuniform sparse 2-D large-FOV optical phased array with a low-power PWM drive. IEEE J. Solid-State Circuits, 54, 1200-1215(2019).
[16] M. Raval, C. V. Poulton, M. R. Watts. Unidirectional waveguide grating antennas with uniform emission for optical phased arrays. Opt. Lett., 42, 2563-2566(2017).
[17] B. Chen, Y. Li, L. Zhang, Y. Li, X. Liu, M. Tao, Y. Hou, H. Tang, Z. Zhi, F. Gao, X. Luo, G. Lo, J. Song. Unidirectional large-scale waveguide grating with uniform radiation for optical phased array. Opt. Express, 29, 20995-21010(2021).
[18] M. Zadka, Y.-C. Chang, A. Mohanty, C. T. Phare, S. P. Roberts, M. Lipson. On-chip platform for a phased array with minimal beam divergence and wide field-of-view. Opt. Express, 26, 2528-2534(2018).
[19] P. Wang, G. Luo, Y. Xu, Y. Li, J. Pan. Design and fabrication of SiN-Si dual-layer optical phased array chip. Photon. Res., 8, 912-919(2020).
[20] S. H. Kim, J. B. You, Y. G. Ha, G. Kang, H. H. Park. Thermo-optic control of the longitudinal radiation angle in a silicon-based optical phased array. Opt. Lett., 44, 411-414(2019).
[21] A. Masood, M. Pantouvaki, G. Lepage, P. Verheyen, J. V. Campenhout, P. Absil, D. V. Thourhout, W. Bogaerts. Comparison of heater architectures for thermal control of silicon photonic circuits. 10th International Conference on Group IV Photonics, 83-84(2013).
[22] X. Tu, T.-Y. Liow, J. Song, X. Luo, Q. Fang, M. Yu, G.-Q. Lo. 50-Gb/s silicon optical modulator with traveling-wave electrodes. Opt. Express, 21, 12776-12782(2013).
[23] S. Y. Siew, B. Li, F. Gao, H. Y. Zheng, G. Q. Lo. Review of silicon photonics technology and platform development. J. Lightwave Technol., 39, 4374-4389(2021).
[24] D. Zhuang, L. Zhagn, X. Han, Y. Li, Y. Li, X. Liu, F. Gao, J. Song. Omnidirectional beam steering using aperiodic optical phased array with high error margin. Opt. Express, 26, 19154-19170(2018).
[25] D. Kwong, A. Hosseini, Z. Yang, R. T. Chen. 1 × 12 unequally spaced waveguide array for actively tuned optical phased array on a silicon nanomembrane. Appl. Phys. Lett., 99, 051104(2011).
[26] C. V. Poulton, Y. Ami, D. B. Cole, M. J. Byrd, R. Manan, V. Diedrik, M. R. Watts. Coherent solid-state LIDAR with silicon photonic optical phased arrays. Opt. Lett., 42, 4091-4094(2017).
[27] D. Pierrottet, F. Amzajerdian, L. Petway, B. Barnes, G. Lockard, M. Rubio. Linear FMCW laser radar for precision range and vector velocity measurements. MRS Proc., 1076, 1076-K04-06(2008).
[28] C. Rogers, A. Y. Piggott, D. J. Thomson, R. F. Wiser, I. E. Opris, S. A. Fortune, A. J. Compston, A. Gondarenko, F. Meng, X. Chen, G. T. Reed, R. Nicolaescu. A universal 3D imaging sensor on a silicon photonics platform. Nature, 590, 256-261(2021).
[29] D. Tan, K. Ikeda, P. C. Sun, Y. Fainman. Group velocity dispersion and self phase modulation in silicon nitride waveguides. Appl. Phys. Lett., 96, 061101(2010).
[30] A. Martin, D. Dodane, L. Leviandier, D. Dolfi, J. Bourderionnet. Photonic integrated circuit based FMCW coherent LiDAR. J. Lightwave Technol., 36, 4640-4645(2018).
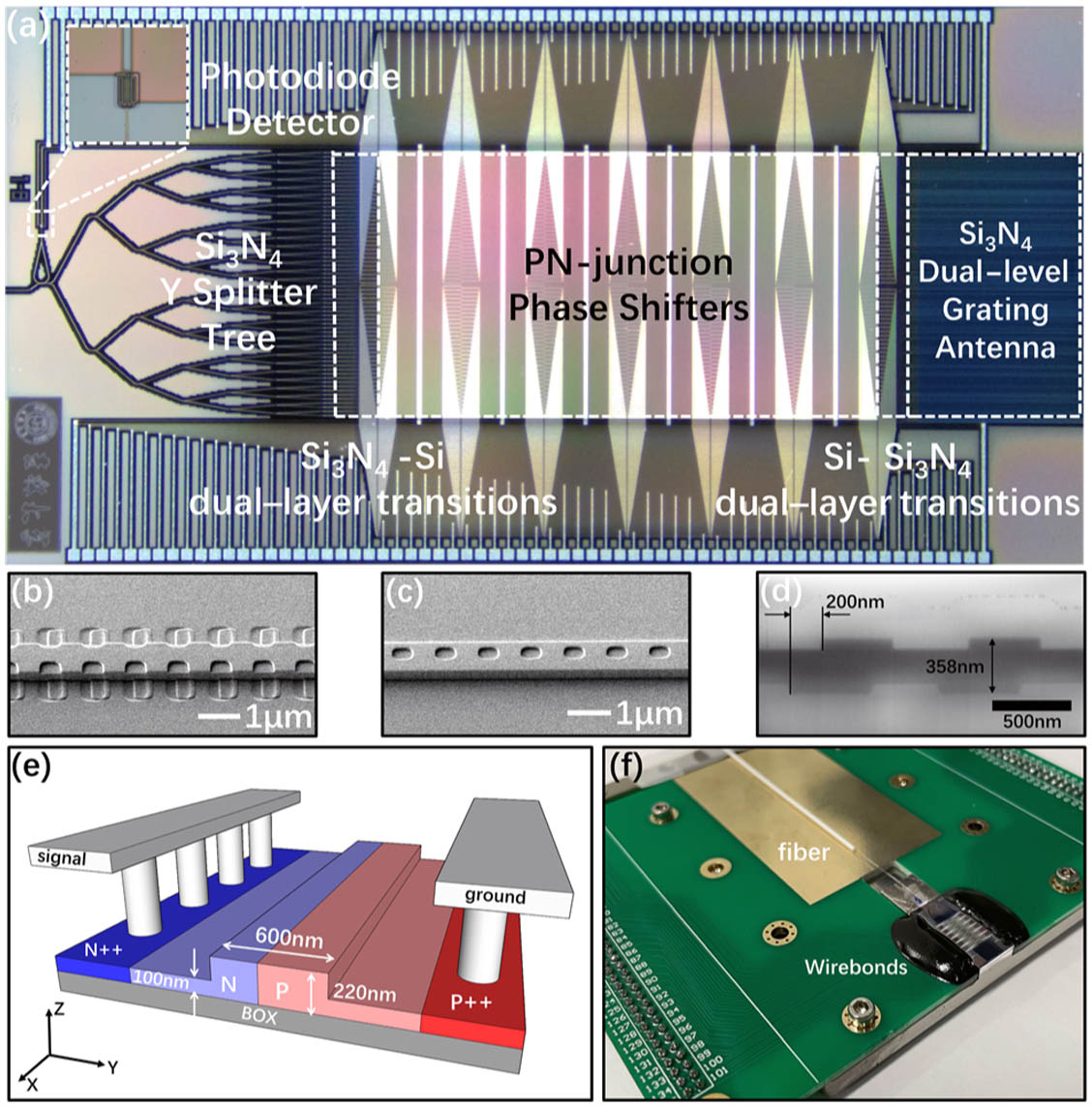
Set citation alerts for the article
Please enter your email address