Abstract
Cavity optomechanics is applied to study the coupling behavior of interacting molecules in surface plasmon systems driven by two-color laser beams. Different from the traditional force–distance measurement, due to a resonant frequency shift or a peak splitting on the probe spectrum, we have proposed a convenient method to measure the van der Waals force strength and interaction energy via nonlinear spectroscopy. The minimum force value can reach approximately 10?15 N, which is 3 to 4 orders of magnitude smaller than the widely applied atomic force microscope (AFM). It is also shown that two adjacent molecules with similar chemical structures and nearly equal vibrational frequencies can be easily distinguished by the splitting of the transparency peak. Based on this coupled optomechanical system, we also conceptually design a tunable optical switch by van der Waals interaction. Our results will provide new approaches for understanding the complex and dynamic interactions in molecule–plasmon systems.1. INTRODUCTION
Surface-enhanced Raman scattering and tip-enhanced Raman scattering (TERS) have been rapidly improved in recent years, providing powerful and reliable analytical tools for studying molecular structures and dynamics [1–10]. Besides, they are also widely exploited in various fields of nano-scale nonlinear optics [11–13] and biomedical applications [14,15]. However, the observations of the large nonlinear effects in the near field of metallic nano-particles [8–10] cannot be explained well by the conventional electromagnetic theory. Recently, Roelli et al. introduced a cavity optomechanics model that accounts for the dynamical nature of the plasmon–molecule interaction, which leads to a nonlinear enhancement of Raman emission, which is not predicted by conventional theories [16,17].
On the other hand, it is well known that the van der Waals force (VF) existing in nonpolar molecules can be considered as a quantum coupling between the instantaneous dipoles. Today, having benefited from the single-molecule force spectroscopy of an atomic force microscope (AFM), the detection of molecular force with sensing range of 1–100 pN has become possible [18–20]. An optical tweezer [21,22], magnetic bead [23], and biomembrane force probe [24] are also used to probe the intermolecular interactions. For the AFM technology, the measuring accuracy depends strongly on the quality of the probe tip and the preparation of samples. The gravity, pollution, nonlinear motion of the tip, or unsuitable samples may remarkably affect the precision in probing. Long-range force and weaker force detection always require higher resolution or new technologies. Recently, Hou et al. reported that two adjacent molecules within van der Waals contact can be distinguished via TERS spectroscopy [25] and the dipole–dipole coupling also can be observed directly by scanning tunneling microscope (STM)-induced luminescence [26]. Those works may indicate the possibility of designing unconventional optical nano-devices used in an ultrasensitive sensor [27,28] and quantum information processor [29,30].
In the present paper, we combine the two research fields. By applying the concepts used in cavity optomechanics, we interpret the surface enhancement as an optomechanical process and investigate the VF interactions in a multi-molecule system. The features of the interactions induced by electromagnetic fluctuations are discussed in detail. Based on this, we propose an exact experimental method to detect the coupling strength and measure the dispersion interaction energy directly via spectroscopy. The traditional intermolecular force measurement techniques are based on the force–distance detection by probe beams [18–22], while we use the ultrasensitive frequency spectrum instead of the displacement spectrum in our scheme. The intermolecular force can be determined at the level of , which is several orders of magnitude smaller than the AFM technology. This technical advancement can make the intermolecular force spectroscopy a widely used tool for the structural and functional investigation of molecules in their native environment. We expect that the method can be achieved in the next few years, and we have explored wide regions of the energy landscapes of ligand–receptor bonds; thus we can reveal a detailed picture of the binding potential. Here we also focus on the phonon-induced transparency (PIT) process in the presence of a tunable VF. We find an interesting VF-controlled optical switch effect; the optical transparency window can be completely shut down and opened by sorely tuning the strength of the VF.
Sign up for Photonics Research TOC. Get the latest issue of Photonics Research delivered right to you!Sign up now
2. THEORY FRAMEWORK
In what follows, we consider a surface plasmon cavity coupled to double molecules with van der Waals dispersion force contact. The typical physical situation is shown in Fig. 1(a). We place the two adjacent molecules in the “hotspot” of the plasmon cavity with simultaneous presence of a strong pump field and a weak probe field. We use the molecules of thiophenol and gold-benzenethiolate (GBT) in simulation. As illustrated in Fig. 1(b), thiophenol has a planar configuration, whereas GBT converges to a perpendicular geometry, where the S–Au bond rotates by 90° to reach the symmetry plane perpendicular to the phenyl ring. The Hamiltonian of the localized plasmon mode with the resonance frequency can be expressed as , where and are bosonic creation and annihilation operators. The molecules can be regarded as harmonic oscillators of frequencies , and the corresponding Hamiltonian is with and the bosonic creation and annihilation operators. We use an interaction term to describe the coherent coupling between the two mechanical oscillators and the electromagnetic cavity mode. The coupling between molecules and plasmon can be quantified by the optomechanical vacuum coupling rate [16]
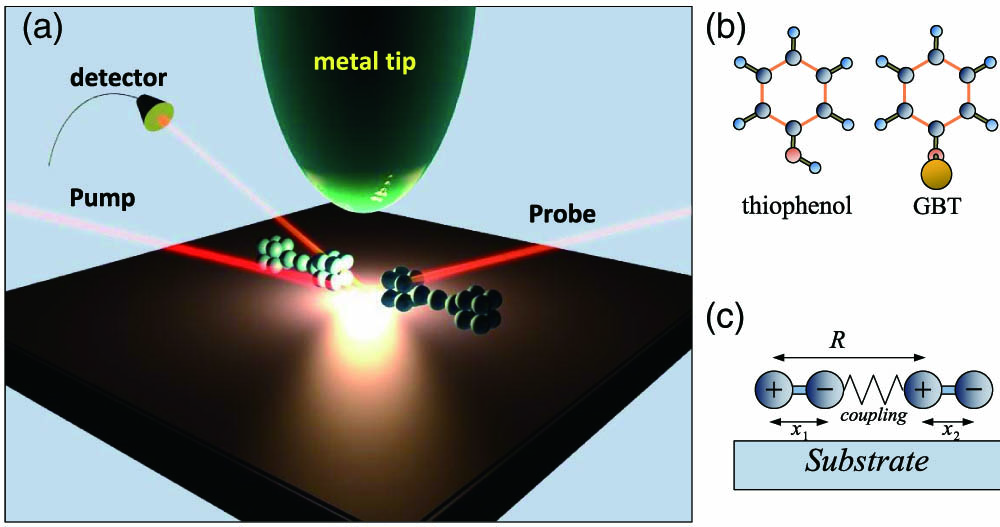
Figure 1.(a) Diagram of surface plasmon cavity optomechanical system driven by a strong pump laser and probed by a weak signal laser. The double molecules reside on the substrate. (b) Molecules of thiophenol and GBT in their lowest energy conformations. (c) Interacting molecules with the intermolecular distance, and and the distances between different charges for the instantaneous dipoles.
Here the polarizability is a material property that depends on the molecular structure and nature of the bonds, is the mode volume of the plasmonic cavity. We use the mass-weighted Cartesian displacement coordinates , where are the effective masses of the vibrational mode of molecules. We then express the vacuum coupling rate as a function of experimentally used parameters (including Gaussian units and wavenumber) as , where is the wavenumber of the molecular vibrational mode in , is the wavelength of the resonance of the plasmon in [nm].
In order to compute , we need to consider the Raman activity , which is a pure molecular property and is independent of the experimental setup. The Raman activities of thiophenol and GBT are experimentally determined as and [16]. From Eq. (1) we can see that the stronger coupling can be achieved by using a smaller plasmonic mode volume. However, we find that the FHWM of the probe transmission spectrum cannot be changed by selecting different optomechanical coupling strengths. The coupling affects only the strength of the transmission peak relatively. The vibrational lifetime determines the FHWM and further determines the resolution in the probe, which provides a high degree of freedom by choosing the volume, frequency, and dissipation rate of the plasmon cavity for the force detections, as we will discuss.
Here the two molecules are modeled by 1D oscillators, the distance between the different charge centers in every molecule is , and is the intermolecular coordinate, as shown in Fig. 1(c). Then the potential energy can be computed as follows [31]: Expanding Eq. (2) in the condition of and working to the lowest order, we can obtain Thus the VF can be regarded as a quantum coupling between the instantaneous dipoles. In the regime of , the effective coupling Hamiltonian can be expressed within rotating wave approximation [32] where and are the effective masses of the two molecules. The coefficient can be defined as the VF coupling strength, which reveals from essence the van der Waals interaction between molecules. The whole system can be considered as an optomechanical system, where two vibrational modes are coupled to a single cavity mode with the coupling rates and , meanwhile interacting through the VF strength . Based on the discussion above, the Hamiltonian of the double oscillators’ cavity optomechanical system in a rotating frame can be expressed as a sum of , and [16,33]: where is probe-cavity detuning, and are related to the laser power by and , respectively.
We define the operator and the detuning of the probe and pump field . According to the Heisenberg equation of motion and the communication relations and . The temporal evolutions of and can be obtained, and the quantum Langevin equations are given by adding the damping terms where is the total energy damping rate of the plasmonic cavity. and are the damping rates of the vibrational mode of the two molecules. is the correlated Langevin noise operator, which has zero mean and obeys the following correlation functions: . The correlators for look like those for as previously listed. The motion of molecules is affected by a thermal bath of the Brownian and non-Markovian stochastic process [34]. The quantum effects on the resonator are only displayed in the limit of a high-quality factor. The resonator mode of molecules is affected by a Brownian stochastic force with zero mean value, and has the correlation function [35] Following standard methods from quantum optics, we derive the steady-state solutions to Eqs. (7)–(9) by setting all the time derivatives to zero: With , , and . We can always rewrite each Heisenberg operator as the sum of its steady-state mean value and a small fluctuation with zero mean value as follows: Inserting these equations into the Langevin equations, one can safely neglect the nonlinear terms such as . Because the driving fields are weak, we will identify all operators with their expectation values and drop the quantum and thermal noise terms [36]. Then the linearized Langevin equations can be written as To solve these equations, we make the ansatz as follows: Substituting Eqs. (18)–(20) into Eqs. (15)–(17), respectively, equating terms with the same time dependence, and working to the lowest order in but to all orders in , we can obtain Solving Eqs. (24)–(29), we obtain with , , , and , and , can be resolved by Eqs. (24) and (27).
The transmission of the probe beam defined as the ratio of the output and input field amplitudes at the probe frequency is then given by [36]
3. NUMERICAL RESULTS AND DISCUSSION
The plasmon mode can be regarded as a bad cavity with quality factor . The resonance frequency of the plasmonic cavity ; thus, we can obtain the plasmonic dissipation rate of [16].
A. Super-Resolution Force Spectroscopy
1. For Identical Molecules
Let us consider the interaction between double GBT molecules placed in the plasmon cavity, which is driven by a strong pump field and a weak field. The fundamental frequencies of the molecular vibrational modes are . We choose the mechanical dissipation rates [16,37]. Then the transmission of the probe beam can be plotted as a function of the probe-cavity detuning with setting and , respectively, in Fig. 2(a). We can obtain three sharp enhanced peaks in turn on the probe spectrum. Without the presence of VF, the peak located at just corresponds to the resonance frequency of GBT. It indicates that those molecules are both vibrating with their common resonance frequency. The blue line in Fig. 2(a) shows that a resonance frequency shift induced by VF can be observed distinctly in the transmission spectrum when . For the case of , the vibrational frequency becomes lower, as depicted by the red line. One mechanism underlying this effect is the following: when the same two molecules couple with the VF, the level of their vibrational dressed state reduces by ; hence the VF-aided enhanced spectrum tends to be shifted to the right. We depicted the schematic of the energy-level diagram in the cavity optomechanical system in Fig. 2(b), where denotes the number states of the cavity photon.
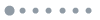
Figure 2.(a) Transmission spectrum of the probe beam as a function of the probe–pump detuning with different van der Waals coupling rates for the same molecules (GBT). We choose ; and . The other parameters are , , , , and . (b) Energy levels of the coupled system corresponding to the transmission peak shift. (c) Linear relationship between frequency shift and coupling rate.
According to the discussion above, the intermolecular interaction between the same two molecules can lead to the redshift in the transmission spectrum and reduce the energy stored in the oscillators. Figure 2(c) shows that the coupling rate has a simple relationship with the frequency shift via . Because the shift distance is exactly equal to the VF coupling rate, we can provide a straight and accurate method to measure the VF strength based on it. The FHWM of the oscillation peak on the transmission spectrum [as shown in Fig. 2(a)] is approximately 0.05 THz. Hence the value of the minimal energy change can be measured down to the accuracy of in this coupling system. According to the equipartition theorem, the root-mean-square (rms) amplitude of the molecules at thermal equilibrium can be expressed as , here is Boltzmann’s constant, and is the effective resonator temperature. We consider the stretching vibration between the benzene ring and the atom of sulfur or gold; thus, we can compute the effective mass as , where and denote the mass of the benzene ring and atom (sulfur and gold). Substituting into Eq. (3), considering , at room temperature (), then we can get the distance , and the minimal measurable force can be estimated by . Today, the most force measurements are mainly based on the sizeable deformation of molecules [18], which are more suitable to adhesive substance and short-range detection. However, compared with AFM, the precision can be increased by 3 to 4 orders of magnitude without the molecular deformation in our scheme.
2. For Distinct Molecules
The transmission spectrum for the VF coupling between two different adjacent molecules exhibits interesting features. Figure 3(a) presents the probe spectra for thiophenol and GBT molecules in plasmon measured under different coupling rates. The two molecules are packed close to each other with VF contact. They have similar chemical structures and nearly equal vibrational frequencies: , . For , it shows a significant transparency peak near the resonant region of the two molecules. Although the two molecules vibrate with different frequencies, the optomechanical vacuum coupling rate of thiophenol is much smaller than GBT [16,37]; hence, there is only one visible enhanced peak on the spectrum, as shown by the black line in Fig. 3(a). When the VF coupling strength increases to , we can see from the blue curve that the transparency peak suffers a significant splitting. The underlying physical mechanism for this phenomenon can be explained by Fig. 3(b) in which the level of vibrational dressed state separates with the distance of . The splitting distance becomes larger with increasing the coupling rate , which is characterized by the red line in Fig. 3(a). Their relationship can be expressed by , as shown in Fig. 3(c). Thus, one can determine the coupling constant by detecting the spectral splitting distance. It also should be noted that the strength of the transparency peak is strongly affected by the intermolecular interaction; thus, we find that the weaker peak of thiophenol can be significantly amplified by the raised VF strength by comparing the blue and red curves in Fig. 3(a). Therefore, the adjacent molecules with approximate vibrational frequencies and similar structural skeletons can be distinguished and identified through the changes of probe spectrum.
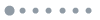
Figure 3.(a) Transmission spectrum of the probe beam as a function of the probe–pump detuning with various van der Waals coupling rates for different two molecules (thiophenol and GBT). We choose ; ; ; and , , , , , . (b) Energy levels of the coupled system corresponding to the enhanced peak splitting. (c) Linear relationship between split distance and coupling rate .
B. Van der Waals Switch
We depict transmission spectra and reflection spectra of the probe field as a function of the probe–pump detuning with the power in Fig. 4. The coupling rate can be modulated by the intermolecular distance , as shown in Eq. (4). The left plot in Fig. 4 exhibits a standard Lorentzian shape and an inverted Lorentzian shape in the reflection and transmission spectra of the probe field with and the VF coupling , which signifies the completely reflected probe beam through the uncoupled molecules. When the molecules get closer to , the reflection spectrum and the transmission spectrum present a completely different behavior, as shown in the right plot in Fig. 4. From the figure, we can see that the transmission is 100%, but the reflection is zero at the resonance. In other words, when pumping the coupled system with a suitable separation, the resonant probe beam will be completely transmitted, while the reflection is totally suppressed, which is opposite to the case of . Due to the fact that the reflection and transmission of the signal beam can be effectively controlled by VF, here we propose a protocol for implementing a VF-switch based on this coupled molecule–plasmon system. By tuning the VF, the probe light can be varied from the transparency regime to the absorption regime or vice versa. Thus the VF-controlled light switch can be shut down and opened via changing the distance of the two molecules. This effect can be considered as a PIT, which is reminiscent of the electromagnetically induced transparency. One mechanism underlying this effect is the following: The coupling between molecules breaks down the symmetry of the PIT interference; thereby the probe absorption window is split into two windows, which yields the VF-induced PIT.
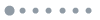
Figure 4.Transmission and reflection spectrum of a signal beam with different range between molecules (thiophenol and GBT) in the case of . Other parameters are same with Fig. 2.
4. CONCLUSION
In summary, by using the optomechanical theory, we have investigated the van der Waals interactions between the adjacent molecules in the surface plasmon cavity under two-color beams. For the identical two molecules, the resonance peak presents a redshift with increasing the coupling strength. It can be used to perform the sensitive measurement of the coupling rate and intermolecular interaction energy. In contrast with AFM technology, the surface plasmon cavity optomechanics display a strong advantage in measuring precision. If the two molecules possess different optomechanical vacuum coupling rates, their transparency peak will suffer a distinct splitting with the interaction force being taken into consideration, which leads to an effective scheme for molecular distinction and also an ultrasensitive measurement for weak force and weak energy. This optical controlled scheme effectively could avoid some errors in the preparation of samples and the nonlinear motions of the probing tips in AFM. According to the PIT, we can design a VF-induced switch by a surface-plasmon-enhanced optomechanical system with two control beams. The physical origin in such coupled systems was explained as four-wave mixing, an important third-order nonlinear process, which is different from the single-beam scheme. The simultaneous presence of a pump field and a probe field generates a radiation pressure force at the beat frequency, which drives the motion of the molecules near its resonance frequency. The driven motion leads to a mechanical sideband on the pump field that can interfere with the probe field. Therefore, a resonantly enhanced nonlinear process can be expected in this system. This scheme can be adapted to study any interactions, which can be regarded as a quantum coupling between the objects. Thus, we expected that the Coulomb’s interaction, the Casimir force between molecules, also can be probed via the cavity optomechanical scheme. However, an experimental challenge will be to precisely control their position at an ultrashort range (). We expected that, within the next few years, this optical domain method can be achieved in experiments.
References
[1] S. Berweger, C. C. Neacsu, Y. Mao, H. Zhou, S. S. Wong, M. B. Raschke. Optical nanocrystallography with tip-enhanced phonon Raman spectroscopy. Nat. Nanotechnol., 4, 496-499(2009).
[2] E. M. van Schrojenstein Lantman, T. Deckert-Gaudig, A. J. G. Mank, V. Deckert, B. M. Weckhuysen. Catalytic processes monitored at the nanoscale with tip-enhanced Raman spectroscopy. Nat. Nanotechnol., 7, 583-586(2012).
[3] J. P. Camden, J. A. Dieringer, Y. Wang, D. J. Masiello, L. D. Marks, G. C. Schatz, R. P. Van Duyne. Probing the structure of single-molecule surface-enhanced Raman scattering hot spots. J. Am. Chem. Soc., 130, 12616-12617(2008).
[4] D. Wang, W. Zhu, M. D. Best, J. P. Camden, K. B. Crozier. Directional Raman scattering from single molecules in the feed gaps of optical antennas. Nano Lett., 13, 2194-2198(2013).
[5] M. D. Sonntag, J. M. Klingsporn, L. K. Garibay, J. M. Roberts, J. A. Dieringer, T. Seideman, K. A. Scheidt, L. Jensen, G. C. Schatz, R. P. Van Duyne. Single-molecule tip-enhanced Raman spectroscopy. J. Phys. Chem. C, 116, 478-483(2012).
[6] J. Steidtner, B. Pettinger. Tip-enhanced Raman spectroscopy and microscopy on single dye molecules with 15 nm resolution. Phys. Rev. Lett., 100, 236101(2008).
[7] R. Treffer, X. Lin, E. Bailo, T. D. Gaudig, V. Deckert. Distinction of nucleobases-a tip-enhanced Raman approach. Beilstein J. Nanotechnol., 2, 628-637(2011).
[8] R. Zhang, Y. Zhang, Z. C. Dong, S. Jiang, C. Zhang, L. G. Chen, L. Zhang, Y. Liao, J. Aizpurua, Y. Lou, J. L. Yang, J. G. Hou. Chemical mapping of a single molecule by plasmon-enhanced Raman scattering. Nature, 498, 82-86(2013).
[9] R. Chikkaraddy, B. Nijs, F. Benz, S. J. Barrow, O. A. Scherman, E. Rosta, A. Demetriadou, P. Fox, O. Hess, J. J. Baumberg. Single-molecule strong coupling at room temperature in plasmonic nanocavities. Nature, 535, 127-130(2016).
[10] W. Zhu, K. B. Crozier. Quantum mechanical limit to plasmonic enhancement as observed by surface-enhanced Raman scattering. Nat. Commun., 5, 5228-5236(2014).
[11] P. Mülschlegel, H. J. Eisler, O. J. F. Martin, B. Hecht, D. W. Pohl. Resonant optical antennas. Science, 308, 1607-1609(2005).
[12] D. R. Ward, F. Hüer, F. Pauly, J. C. Cuevas, D. Natelson. Optical rectification and field enhancement in a plasmonic nanogap. Nat. Nanotechnol., 5, 732-736(2010).
[13] S. Gwo, C. Y. Wang, H. Y. Chen, M. H. Lin, L. Sun, X. Li, W. L. Chen, Y. M. Chang, H. Ahn. Plasmonic metasurfaces for nonlinear optics and quantitative SERS. ACS Photon., 3, 1371-1384(2016).
[14] X. M. Qian, S. M. Nie. Single-molecule and single-nanoparticle SERS: from fundamental mechanisms to biomedical applications. Chem. Soc. Rev., 37, 912-920(2008).
[15] J. Prinz, C. Heck, L. Ellerik, V. Merk, I. Bald. DNA origami based Au-Ag-core-shell nanoparticle dimers with single-molecule SERS sensitivity. Nanoscale, 8, 5612-5620(2016).
[16] P. Roelli, C. Galland, N. Piro, T. J. Kippenberg. Molecular cavity optomechanics as a theory of plasmon-enhanced Raman scattering. Nat. Nanotechnol., 11, 164-169(2016).
[17] M. K. Schmidt, J. Aizpurua. Nanocavities: optomechanics goes molecular. Nat. Nanotechnol., 11, 114-115(2016).
[18] T. Hugel, M. Seitz. The study of molecular interactions by AFM force spectroscopy. Macromol. Rapid Commun., 22, 989-1016(2001).
[19] S. Kawai, A. S. Foster, T. Björkman, S. Nowakowska, J. Björk, F. F. Canova, L. H. Gade, T. A. Jung, E. Meyer. Van der Waals interactions and the limits of isolated atom models at interfaces. Nat. Commun., 7, 11559(2016).
[20] S. P. Jarvis, M. A. Rashid, A. Sweetman, J. Leaf, S. Taylor, P. Moriarty, J. Dunn. Intermolecular artifacts in probe microscope images of C60 assemblies. Phys. Rev. B, 92, 241405(2015).
[21] L. Béguin, A. Vernier, R. Chicireanu, T. Lahaye, A. Browaeys. Direct measurement of the van der Waals interaction between two Rydberg atoms. Phys. Rev. Lett., 110, 263201(2013).
[22] G. Ranjit, M. Cunningham, K. Casey, A. A. Geraci. Zeptonewton force sensing with nanospheres in an optical lattice. Phys. Rev. A, 93, 053801(2016).
[23] S. B. Smith, L. Finzi, C. Bustamante. Direct mechanical measurements of the elasticity of single DNA molecules by using magnetic beads. Science, 258, 1122-1126(1992).
[24] C. Gourier, A. Jegou, J. Husson, F. Pincet. A nanospring named erythrocyte. The biomembrane force probe. Cell. Mol. Bioeng., 1, 263-275(2008).
[25] S. Jiang, Y. Zhang, R. Zhang, C. Hu, M. Liao, Y. Lou, J. Yang, Z. Dong, J. G. Hou. Distinguishing adjacent molecules on a surface using plasmon-enhanced Raman scattering. Nat. Nanotechnol., 10, 865-869(2015).
[26] Y. Zhang, Y. Luo, Y. Zhang, Y. J. Yu, Y. M. Kuang, L. Zhang, Q. S. Meng, Y. Luo, J. L. Yang, Z. C. Dong, J. G. Hou. Visualizing coherent intermolecular dipole-dipole coupling in real space. Nature, 531, 623-627(2016).
[27] J. A. Scholl, A. L. Koh, J. A. Dionne. Quantum plasmon resonances of individual metallic nanoparticles. Nature, 483, 421-427(2012).
[28] S. Nie, S. R. Emory. Probing single molecules and single nanoparticles by surface-enhanced Raman scattering. Science, 275, 1102-1106(1997).
[29] K. J. Savage, M. M. Hawkeye, R. Esteban, A. G. Borisov, J. Aizpurua, J. J. Baumberg. Revealing the quantum regime in tunnelling plasmonics. Nature, 491, 574-577(2012).
[30] C. Wang, Y. Y. Gao, P. Reinhold, R. W. Heeres, N. Ofek, K. Chou, C. Axline, M. Reagor, J. Blumoff, K. M. Sliwa, L. Frunzio, S. M. Girvin, L. Jiang, M. Mirrahimi, M. H. Devoret, R. J. Schoelkopf. A Schrödinger cat living in two boxes. Science, 352, 1087-1091(2016).
[31] C. Kittel. Introduction to Solid State Physics, 43-44(2005).
[32] K. R. Brown, C. Ospelkaus, Y. Colombe, A. C. Wilson, D. Leibfried, D. J. Wineland. Coupled quantized mechanical oscillators. Nature, 471, 196-199(2011).
[33] P. C. Ma, J. Q. Zhang, Y. Xiao, M. Feng, Z. M. Zhang. Tunable double optomechanically induced transparency in an optomechanical system. Phys. Rev. A, 90, 043825(2014).
[34] C. Gardiner, P. Zoller. Quantum Noise: A Handbook of Markovian and Non-Markovian Quantum Stochastic Methods with Applications to Quantum Optics, 425(2000).
[35] V. Giovannetti, D. Vitali. Phase-noise measurement in a cavity with a movable mirror undergoing quantum Brownian motion. Phys. Rev. A, 63, 023812(2001).
[36] S. Weis, R. Rivière, S. Deléglise, E. Gavartin, O. Arcizet, A. Schliesser, T. J. Kippenberg. Optomechanically induced transparency. Science, 330, 1520-1523(2010).
[37] C. Humbert, O. Pluchery, E. Lacaze, A. Tadjeddine, B. Busson. A multiscale description of molecular adsorption on gold nanoparticles by nonlinear optical spectroscopy. Phys. Chem. Chem. Phys., 14, 280-289(2012).