Author Affiliations
1Guangdong University of Education, School of Physics and Information Engineering, Guangzhou, China2University of Delaware, Department of Electrical and Computer Engineering, Newark, Delaware, United States3University of California, Department of Electrical Engineering and Computer Science, Irvine, California, United States4Jazan University, Faculty of Science, Department of Physics, Jazan, Saudi Arabia5Ibb University, Faculty of Science, Department of Physics, Ibb, Yemen6Institute of Energy and Climate Research, Materials Synthesis and Processing (IEK-1), Forschungszentrum Jülich GmbH, Jülich, Germany7University of Science and Technology of China, Hefei National Laboratory for Physical Sciences at the Microscale, Hefei, China8Riphah International University Faisalabad, Department of Physics, Faisalabad, Pakistanshow less
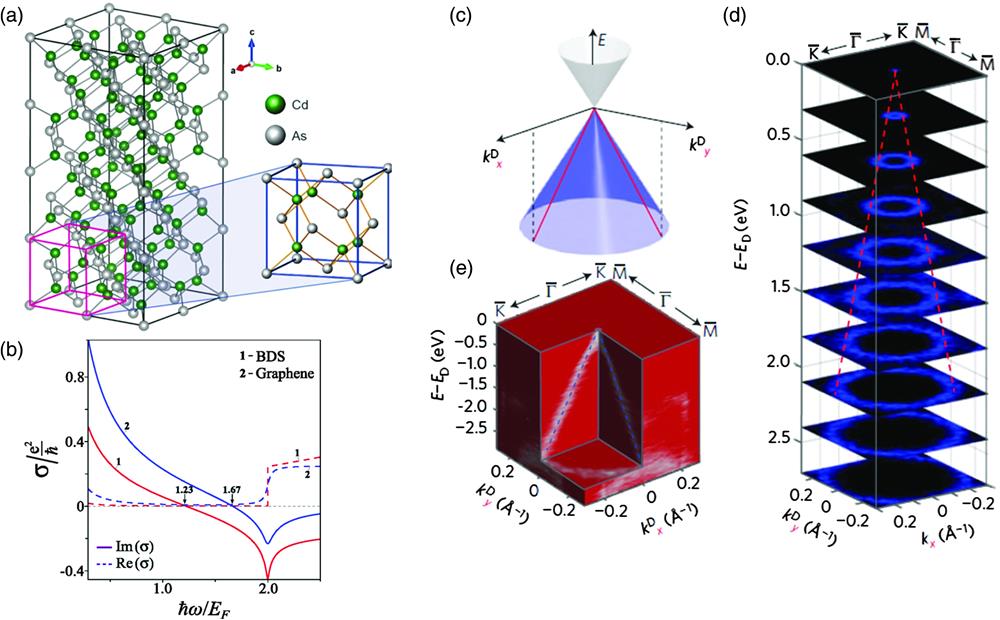
Fig. 1. Crystal structure, projections of the 3D Dirac fermions, and refractive index of 3D DSM. (a)
nonprimitive tetragonal unit cell is made up of 24 slightly deformed antifluorite cells with two cadmium vacancies. 96 cadmium atoms and 64 arsenic atoms are contained in this cell. (b) The real and imaginary components of dynamic conductivity in 3D DSMs. (c) Schematic representation of the projected Dirac cone into the (
,
,
) space reconstructed from experimental values. The red lines show the linear dispersions along the
and
axes. (d) Stacking plots of constant-energy contours at different binding energies reveal the structure of the Dirac cone band. The red dotted lines serve as visual cues for the dispersions and intersect at the Dirac point. (e) A three-dimensional intensity plot of the photoemission spectrum at the Dirac point, demonstrating identical cone-shaped dispersion to that in (c). The figure is reproduced with the permission of (a) Ref.
34 © 2018 American Physical Society, (b) Ref.
35 © 2016 American Physical Society, and (c)–(e) Ref.
3 © 2018 Nature Publishing Group.
Fig. 2. Experimental methodologies to grow
nanostructures, thin films, single crystals, and fabrication of
-based devices. (a) Schematic diagram of nucleation and growth process of
nanostructures by PVD. (b) Flow diagram of CVD growth of nano-wires. (c) Display of
thin films based device fabricated by MBE, showing excellent flexibility. (d) Schematic diagram of pulsed laser deposition technique to grow
thin films. (e)
based heterostructure device fabricated by the CVD method. (f) SSVG system to grow single crystal
. The figure is reproduced with the permission of (a) Ref.
44 © 2015 American Physical Society, (b) Ref.
50 © 2021 Springer Nature, (c) Ref.
46 © 2019 American Physical Society, (d) Ref.
51 © 1984 Elsevier, (e) Ref.
52 © 2021 Wiley-VCH GmbH, and (f) Ref.
53 © 2015 Nature Publishing Group.
Fig. 3. OHG in 3D DSM
. (a) Schematic demonstration of the generation of HHG from 3D DSM thin film. (b) Room temperature HHG in
film and air. (c) Theoretically estimated HHG in a 3D DSM thin film when a linearly polarized pulse with a central frequency of 1 THz and a peak field intensity of 10 MV/m generates harmonics up to the 31st order and beyond with energy conversion efficiency far over
. (d) A comparison of the THG signal among the
, glass,
, and graphene. The figure is reproduced with the permission of (a) Ref.
33 © 2021 Nature Publishing Group, (b) Ref.
32 © 2020 Nature Publishing Group, (c) Ref.
94 © 2020 American Physical Society, and (d) Ref.
93 © 2020 AIP Publishing.
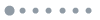
Fig. 4. Plasmonics in 3D DSM. (a) The variation of optical conductivity with a THz frequency in a
thin film. (b) Stripes of
thin film with a transmission spectrum demonstrating plasmonic resonances and an inset illustrating the transmission line model for transmission through a thin film (
), where intraband conductivity can be modeled as series resistance,
, and kinetic inductance,
, which provides the Drude dispersion. (d) The distribution of the electric field at the
direction in a proposed PIT system at frequency of 1.335 THz. (e) Transmission spectra of a patterned stripe array showing plasmonic resonances for a
thin film whose dimensions are presented in the inset of this figure. (f) A comparison of surface plasmon propagation lengths between different plasmonic waveguides. The figure is reproduced with the permission of (a)–(c) and (e) Ref.
100 © 2019 American Chemical Society, (d) Ref.
105 © 2017 Optical Society of America, and (f) Ref.
19 © 2019 AIP Publishing.
Fig. 5. Ultrafast photocarrier dynamics in 3D DSM:
. (a) Schematic representation of the excitation and relaxation of electrons and holes from process 1 to 5 (1. before excitation, 2. immediately after excitation, 3. high-temperature thermal distribution, 4. partially cooled distribution with inverted populations, 5. a partially cooled, noninverted distribution). (b) The
sample structure is shown schematically with the illustration of optical pump THz probe spectroscopy. (c) The modulation depth (MD) at 1.0 THz versus pump fluences of
thin film at room temperature. The figure is reproduced with the permission of (a) Ref.
133 © 2017 AIP Publishing, (b) Ref.
113 © 2019 AIP Publishing, and (c) Ref.
23 © 2021 Wiley-VCH GmbH.
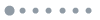
Fig. 6. Perfect absorption using 3D DSM and some other photonic properties. (a) A schematic of the perfect absorber composed of a patterned 3D DSM film, the dielectric layer, and a metal reflector. (b) Calculated electric field distribution of a crossed shape absorber composed of 3D DSM. (c) Simulated absorption of a metamaterial absorber based on 3D DSM. (d) Measured reflectivity of
at various temperatures. The reflectance of gold was used to normalize all measurements. (e) and (f) Static photoemission electron microscopic (PEEM) image of fabricated
nanostructures excited by a linear-polarized 410 nm laser pulse (e) and circularly polarized light (f). The polarization is manifested by the red arrow. (g) Reflection amplitude of a coding unit cell of 0 and 1 of metasurface made with 3D DSM. The figure is reproduced with the permission of (a) Ref.
154 © 2019 Optical Society of America (OSA), (b) Ref.
158 © 2021 MDPI, (c) Ref.
156 © 2018 Optical Society of America (OSA), (d) Ref.
16 © 2020 Wiley-VCH GmbH, (e) and (f) Ref.
164 © 2022 Wiley-VCH GmbH, and (g) Ref.
165 © 2022 Elsevier.
Fig. 7. Schematic shows the potential applications that can generate with the photonics of 3D DSM .
Material | Preparation method | Precursor/target | Growth temperature | Substrate | Reference | thin film | PLD | Mixture of 6N5 Cd and 7N5 As shots target | Room temperature | | Ref. 45 | thin film | PLD | Single crystal target | Room temperature | Fused quartz plates | Ref. 51 | nanostructures | PVD | powder | 650°C | | Ref. 44 | thin film | MBE | bulk | 170°C | Mica | Ref. 21 | thin film | MBE | target | 140°C | CdTe | Ref. 60 | thin film | MBE | target | 150°C to 170°C | GaAs | Ref. 49 | thin film | MBE | target | 180°C | GaAs (111)B | Ref. 61 | nanostructures | CVD/ vapor solid mechanism | powder | 760°C | Si | Ref. 47 | nano-belts | CVD | powder | 760°C | Quartz | Ref. 52 | microbelts | CVD | powder | 760°C | Si | Ref. 58 | submicron wires | CVD | powder (6N pure) | Upstream: 650°C Downstream: 350°C | coated with L / ethanol solution | Ref. 62 | single crystals | CVD | Cd and As powders | 825°C | — | Ref. 63 | single crystals | SSVG | Stoichiometric mixture of Cd and As elements | 850°C | — | Ref. 53 |
|
Table 1. Growth conditions for Cd3As2 thin films, nanostructures, and single crystals for various growth techniques.
Sr. No | Fabrication technique | Advantage | Disadvantage | Reference | 1 | Molecular beam epitaxy | 1. Deposition is carried out in ultrahigh vacuum (up to ). | 1. It causes poor crystallinity of material () due to low growth temperature. | Refs. 45, 46, 64, 65 | 2. High-quality large-area epitaxial thin films can be grown with controlled thickness. | 3. Good capacity to produce heterostructures and superlattices with abrupt interfaces. | 2 | Pulse laser deposition | 1. Suitable for the growth of materials () with high melting points, complicated stoichiometry, and sandwich architectures. | 1. It causes nonuniformity of particulate size across the surface of films due to the presence of molten material in the ablated material. | Refs. 45, 55, 65–67 | 2. Can be operated in both inert and ultrahigh vacuum. High deposition rates and takes short time for deposition. | 2. There is a possibility of splashing and formation of microsized island on the surface of deposited film. | 3 | Chemical vapor deposition | 1. Feasible to synthesize both thin films and nanostructures. | 1. Limited capacity to produce heterostructures and superlattices of with abrupt interfaces. | Refs. 43, 52, 58 | 2. Versatile, adaptable, compatible, simplest, reproducible, productive, and cost effective technique. | 2. Film thickness is not easy to control. | 3. In CVD, deposition at high temperatures cause stresses in the thin films and limits the choice of substrates. | 4 | Physical vapor deposition | 1. It is suitable to synthesize both thin films and nanostructures. | 1. Low deposition rates. | Refs. 44, 45, 56 | 2. It involves both thermal heating and kinetic ejection of target material. | 2. Difficulty in evaporating and formation of alloys thin films. | 3. Film thickness control is easy and it is environment friendly and easy to use. | 3. It is extremely difficult to control stoichiometry of thin films. | 5 | Self-selecting vapor growth | 1. Suitable to grow single crystals. | 1. The growth process for is very slow and deposition can take place a week to form. | Refs. 53, 59 | 2. It involves little variation in temperature between the evaporation and deposition portions. | 2. The crystal shape of is not controllable. | 3. Scale-up beyond has not been demonstrated. |
|
Table 2. The advantages and disadvantages of various fabrication techniques for the fabrication of Cd3As2.
Nonlinear material | Reference | / | Phase control | Advantage | Disadvantage | Traditional bulk material () | Refs. 85, 86 | | No | Commercially available | Weak nonlinearity | Plasmonic nanostructures (gold) | Refs. 86–88 | | Yes | Strong field enhancement | High losses | Dielectric nanostructures | Refs. 89 and 90 | | Yes | Low losses | Moderate field enhancement | 2D materials thin films | Refs. 69, 91, 92 | | Yes | 1. Tunable | 1. Due to atomic thickness the light–matter interaction is weak. | 2. Easily integrated with other bulk structures | 2. Making nanostructures is difficult | thin film | Ref. 93 | | Yes | 1. Tunable | Need to be explored (Very few experimental findings and minimal understanding) | 2. Extendable to other structures | 3. Easy to make nanostructures |
|
Table 3. Comparison between Cd3As2 nonlinear efficiency with other materials including advantages and disadvantages.