
- Advanced Photonics
- Vol. 5, Issue 4, 046010 (2023)
Abstract
1 Introduction
Light waves, as well as universal waves, encounter spatial, temporal, spectral, and polarization distortions when propagated through inhomogeneous media, which is detrimental for many applications, such as optical manipulation, imaging, and communication. In a scattering medium, ballistic photons are exponentially attenuated with respect to its propagation depth, while the scattered light gives rise to speckle patterns, i.e., random interference.1 The scattering process is deterministic, and the information is not lost within the decorrelation time. The wavefront-shaping method is a powerful tool to offset the scattering effect and correct wave distortions.2 Over the last decade, wavefront-shaping methods have shown great capability to achieve refocusing,3,4 imaging,5
Nonlinearity is of great significance and widely used in many applications, including biological sensing,9 nonlinear imaging,10 and phototherapy.11 Controlling light in scattering media with nonlinearity is of fundamental and practical significance. The combination of nonlinearity and wavefront-shaping methods provides more controllable dimensions and advantages. When the scattering medium involves quadratic nonlinearity, a speckle pattern at the harmonic frequency can be generated due to scattering. Manipulation of nonlinear signals in nonlinear scattering media is quite significant and meaningful, which has important applications in controlling multidimensional spectral–spatiotemporal interactions,12,13 light focusing in multimode fibers,14 nonlinear imaging,15 and nonlinear photodynamic therapy.16 Wavefront-shaping techniques are also widely used in manipulating nonlinear scattering signals17,18 or investigating nonlinear properties of scattering media.19 However, most implementations are based on feedback mechanisms and only perform next-generation optimization based on the feedback signal of the current generation. Although the focusing of scattered light can be achieved at a submicrosecond time scale by the process of field self-organization inside a multimode laser cavity,20 the focusing position depends on the position of the retroreflecting target. On the contrary, the scattering-matrix (SM) method,21,22 including transmission/back-SM method, can generate arbitrary output and minimize the optimization time, suitable for applications in dynamic scattering environments.4 It is achieved by continuously projecting a set of predefined phase masks to the scattering medium and solving the inverse problem. In addition, since the SM connects the input and output light, this method can also be used to describe the mesoscopic properties of the scattering medium, such as the memory effect23 and transmission eigenchannels.24,25 The conventional SM becomes inadequate when the scattering medium also exhibits nonlinearity. The SM method in nonlinear optics would provide a significant method for studying physical properties of nonlinear scattering media, which is fundamentally important for nonlinear imaging, nonlinear signal manipulation, quantum information processing in complex environments, lithography, and molecular spectroscopy.
In this paper, we experimentally determine the SM of a scattering medium with quadratic nonlinearity via the four-phases interferometric method (FPIM). We reveal the physical mechanism of nonlinear process in the nonlinear scattering medium and provide a new way for manipulating nonlinear signals with wavefront shaping based on the SM. The validity and accuracy of the SM method is proven by refocusing of single-spot, double-spot nonlinear signals and dynamic focusing such as scanning along predefined trajectories combined with the optical phase conjugation technique. Moreover, we investigate the statistic properties of the matrix element and the singular value of the SM of the nonlinear scattering medium. The results show that this SM follows the random matrix theory (RMT).
Sign up for Advanced Photonics TOC. Get the latest issue of Advanced Photonics delivered right to you!Sign up now
2 Principle
To selectively focus a scattering signal through a scattering medium, the input–output relation between each input mode and output mode needs to be determined. For a scattering medium with quadratic nonlinearity, if the input field
The schematic of nonlinear signal focusing via wavefront shaping based on SM is shown in Fig. 1. The nonlinear signal is monitored in the backscattering configuration. Without shaping the wavefront of the input field
Figure 1.Schematic of controlling nonlinear light in scattering medium via the scattering-matrix method. (a) Generation of nonlinear speckle pattern without shaping the wavefront of the input fields. (b) Nonlinear signals generation and scattering process in LN powder. (c) Intensity of nonlinear signal for a variable polarization of input field
3 Experimental Results
Experimental measurement of the SM of the nonlinear scattering medium is illustrated in Fig. 2. The experiment detail is depicted in Fig. 2(a). Two linearly polarized continuous wave lasers at the wavelengths of
Figure 2.The SM measurement process. (a) Experimental setup. L1 to L5, lenses,
To show the validity of the SM measurement, we present single-spot and double-spot focusing of a nonlinear signal on different positions at the region of interest (ROI). The CCD is divided into 16 superpixels × 16 superpixels via pixel binning, corresponding to
Figure 3.Reconfigurable focusing of nonlinear signals via wavefront-shaping method based on SM. (a) Measured SM that connects the input modes (horizontal axis) and output nonlinear modes (vertical axis). Hue and brightness represent phase and amplitude, respectively. (b) Calculated phase patterns for focusing nonlinear signals on different positions of the ROI with superpixel coordinates (3, 5) (red); (9, 9) (blue); (6, 3), and (6, 7) (green, double spots). (c) Focal spots located at different subregions of the ROI with the corresponding optimized phase patterns. (d) Intensity cross section of the nonlinear focal spots located on different subregions of the ROI (red, green, and blue curves).
The single-spot and double-spot focusing experiments demonstrate the validity of the SM method; that is, the SM effectively characterizes the relationship between the nonlinear output field and the input pump field. In a multiple scattering regime, statistical properties of the SM of linear scattering medium follow the RMT.21 Theoretically, the measured SM of nonlinear scattering medium is also related to the SM, and similarly, we show that the statistical properties of the SM also follow the RMT. This can be inferred from the fact that a random system dominated by multiple scattering can be described by a random matrix of independent identically distributed entries of Gaussian statistics under certain conditions.21Figures 4(a) and 4(b) show the statistical distributions of the real and imaginary parts of the SM, respectively. Both obey the Gaussian statistics of the same distribution.
Figure 4.Statistical properties of the SM: distribution of the (a), (b) real and imaginary parts of the measured SM and (c) the normalized singular value of the SM. The blue dashed line represents the singular value distribution from the quarter-circle law. Inset: normalized singular value of the matrix after removing the neighboring element. (d) Normalized amplitude profile of the focusing operator
Singular value decomposition (SVD) is a powerful tool to analyze the SM, which has been used to identify transmission eigenchannels25 and for selective focusing.26,27 For a multiple scattering medium, statistical distribution of the singular value of the SM follows the quarter-circle law.21 To facilitate comparison with the theoretical singular value of RMT, the singular value
Dynamic control of focusing light is extremely important in many fields, such as scanning near-field optical microscopy28 and atomic force microscopy.29 Realization of all-optical signal scanning through scattering media would further improve the imaging resolution.30 We experimentally realize point-by-point scan of nonlinear focus along predefined trajectories. Figure 5(a) shows the predefined S-shape scan trajectory of the nonlinear focus. The direction of the white arrow represents the scanning direction. The nonlinear focus of each position in the scanning path is shown in Fig. 5(b). The intensity and size of the focal spot maintain good uniformity. The nonlinear focus spot moves along the predefined scan path when the phase pattern on the SLM is defined, and the scanning speed of the nonlinear focus is 60 Hz, which is only limited by the frame rate of the SLM. The actual scan trajectory of the focus is shown in Fig. 5(c). Other predefined and actual scan trajectories of the nonlinear focus with the shape of the letters “J,” “T,” and “U” are shown in Figs. 5(d) and 5(e), respectively. The results provide a new solution for realization of high spatial resolution point-by-point scanning microscopic imaging, particles trapping31 through high-scattering media.
Figure 5.Nonlinear focusing along predefined trajectories. (a) Predefined scan trajectory in the shape of the letter “S” of the nonlinear focus. The scanning direction is marked by the arrow. (b) Nonlinear focus of each position in the scan path at different times. (c) Actual trajectory of the nonlinear focus in the shape of the letter “S.” (d) Predefined scan trajectories in the shape of the letters “J,” “T,” and “U.” (e) Actual trajectory of the nonlinear focus in the shape of the letters “J,” “T,” and “U.”
4 Discussion
We have presented an approach for measuring the SM in the scattering materials with nonlinearity. Compared with feedback algorithm, the SM-based WS method can greatly improve the speed. The feedback algorithm takes a great deal of time during the optimization. However, the SM method uses a set of predefined measurement patterns as phase masks, which allows the SLM to display all phase masks at its maximum frame rate without feedback. For
Theoretically, the PBR of a nonlinear focus equals that in a linear case and is expected to be
The establishment of the SM also provides a new and powerful method for nonlinear signal information processing in a strongly scattering environment. The establishment of the SM helps us to further understand the scattering characteristics in the nonlinear scattering medium and further expands its application scenarios. By utilizing the nanoscale probe,33 the scattering nonlinear signals may be expected to focus inside a scattering medium.34 Combining the WS technique with the point-spread function engineering method,35 it is expected that the scattering nonlinear signals can be focused into a variety of novel beams. Polarization control of nonlinear scattering signals is expected to be achieved by measuring the vector SM.8 In addition, the SM method may be pioneering new ways for nonlinear imaging through scattering media. As a proof-of-principle experiment, we only present the measurement of the SM in the SFG process. Such SM method can be extended to other nonlinear processes, such as second-harmonic generation, third-harmonic generation, four-wave mixing, and stimulated Raman scattering with proper configurations. The SM proposed may also provide a solution for quantum information restoration and long-distance quantum imaging in a strong scattering environment. Furthermore, the proposed SM method is expected to be applied to the field of micro- and nanophotonics in the future. Currently, many metasurface-based nonlinear wavefront controls have been proposed.36,37 However, most of these nonlinear metasurfaces are static and cannot modulate the nonlinear signals dynamically. By combining metasurface-based SLMs,38 SM measurements of static nonlinear metasurfaces can be realized to achieve dynamic modulation of nonlinear signals.
5 Conclusion
We have demonstrated nonlinear harmonic wave manipulation in a nonlinear scattering medium via the SM method. To illustrate its validity and accuracy, we have realized the nonlinear signal single-spot, multispot focusing, and dynamic focusing like scan along predefined trajectories. Moreover, we have shown that the statistical properties of the SM still follow the RMT. Our work provides a significant and meaningful tool for nonlinear signal recovery, nonlinear microscopic imaging and detection, microscopic object tracking through scattering media, and complex environment quantum information processing.
Fengchao Ni is a PhD student in the School of Physics and Astronomy at Shanghai Jiao Tong University (SJTU). He received his bachelor’s degree from the School of Information and Optoelectronic Science and Engineering at South China Normal University in 2019. His research focuses on nonlinear optics.
Haigang Liu received his PhD from Shanghai Jiao Tong University. He has been working as an assistant researcher since July 2020. His research focuses on nonlinear optics, micro-nano photonics, information optics and other fields to achieve light field manipulation research.
Yuanlin Zheng is a professor in the School of Physics and Astronomy at SJTU. He obtained his PhD in optics from SJTU in 2013 and subsequently worked as a postdoctoral, assistant, and associate researcher in LAPMP at SJTU. His research focuses on nonlinear optics and integrated photonics for light-matter interaction and light manipulation applications.
Xianfeng Chen is a distinguished professor in the School of Physics and Astronomy at SJTU. He obtained his PhD in physics at SJTU in 1999. He is the executive director of the Research Center for Optical Science and Engineering at SJTU. His current research focuses on advanced photonics materials and devices, nonlinear optics, nanophotonics, quantum optics, and ultrafast optics.
References
[1] J. W. Goodman. Some fundamental properties of speckle. J. Opt. Soc. Am., 66, 1145-1150(1976).
[5] S. Popoff et al. Image transmission through an opaque material. Nat. Commun., 1, 81(2010).
[29] M. Krieg et al. Atomic force microscopy-based mechanobiology. Nat. Rev. Phys., 1, 41-57(2019).
[31] C. Min et al. Focused plasmonic trapping of metallic particles. Nat. Commun., 4, 2891(2013).
[32] A. M. Paniagua-Diaz, W. L. Barnes, J. Bertolotti. Wavefront shaping to improve beam quality: converting a speckle pattern into a Gaussian spot(2021).
[36] M. L. Tseng et al. Vacuum ultraviolet nonlinear metalens. Sci. Adv., 8, eabn5644(2022).
[42] R. Sapienza et al. Anisotropic weak localization of light. Phys. Rev. Lett., 92, 033903(2004).
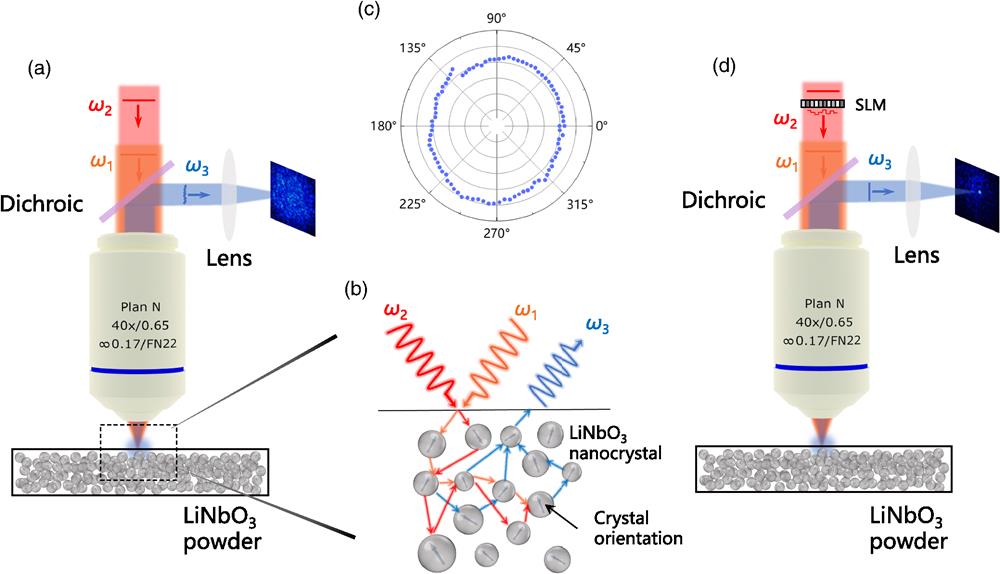
Set citation alerts for the article
Please enter your email address